Measurement of the Dark Matter Velocity Dispersion with Dwarf Galaxy Rotation Curves ()
1. Introduction
We consider non-relativistic dark matter as a classical (non-degenerate) gas, with negative chemical potential, as justified in [1] [2]. Let
be the root-mean-square velocity of non-relativistic dark matter particles in the early universe at expansion parameter
(normalized to
at the present time). As the universe expands it cools, so
is proportional to
(if dark matter collisions, if any, do not excite particle internal degrees of freedom, see digression below). Therefore
(1)
is an adiabatic invariant.
is the density of dark matter, and
. (We use the standard notation in cosmology, and the parameters, as in [3]. Throughout, the subscript h refers to dark matter, and the subscript b will refer to baryons, i.e. gas and stars.) The purpose of this study is to obtain
from the rotation curves of dwarf galaxies dominated by a dark matter halo, and with a thin disk of rotating gas and stars (that allows the measurement of the galaxy rotation curves). If the measured
turns out to be consistent with zero, we say that dark matter is cold. If
turns out to be significantly greater than zero, we say that dark matter is warm (if already non-relativistic when densities of radiation and matter are equal).
Our prediction, which needs confirmation by data, is this. Consider a free observer in the center of a spherically symmetric density perturbation in the early universe. This observer feels no gravity, and “sees” dark matter expand adiabatically, reach turn-around, and contract adiabatically into the core of a galaxy (note that if dark matter is warm the galaxy forms a core adiabatically [4]). Since
is an adiabatic invariant, we predict that
has the same value in the core of all relaxed galaxies, and is of cosmological origin. There are two subtleties to this argument. One is that what we are able to measure is
, where
is a dark matter rotation correction factor, with
.
if the dark matter particle orbits are radial, while
if the dark matter particles approach circular orbits.
is largely unknown. The second issue is “phase space dilution” due to non-adiabatic processes, e.g. galaxy interactions and mergers with relaxation, or the formation of galaxies with relaxation and “virialization” in the cold dark matter scenario. To obtain
, we will have to deal with these two issues.
Digression: Due to the subtleties of self-gravitating dark matter, it is convenient to recall how (1) can be derived for non-relativistic particles. 1) Consider an expanding universe. The velocity of a free particle with respect to that comoving observer that is momentarily at the position of the particle, is proportional to
. To obtain this result use Hubble’s law with
. 2) The adiabatic expansion of a collisional, or collisionless, classical noble gas satisfies
, with
. Since
, and
,
. 3) To define “orbitals”, i.e. volumes in phase space, apply periodic boundary conditions to an expanding box. The momentum of each orbital satisfies
(valid for ultra-relativistic or non-relativistic particles). So
is constant if the mean numbers of particles per orbital remain constant. 4) To prove that a free particle has momentum
use the argument in 1) with a Lorentz transformation. 5) The Collisionless Boltzmann Equation expresses that the number of particles per unit phase-space volume, as seen by any free particle, is constant (in the approximation of being collisionless and conserved), which again leads to
. 6) The relation
can also be obtained from the ideal noble gas Sackur-Tetrode entropy = constant.
We have already carried out this measurement with 46 spiral galaxies in the “Spitzer Photometry and Accurate Rotation Curves” (SPARC) sample, obtaining a distribution of
with mean 0.915 km/s, and standard deviation 0.332 km/s [5], see Figure 1. This small relative standard deviation is noteworthy, and, considering that the galaxies span three orders of magnitude in luminosity, and considering the uncertainties from rotation and relaxation, gives support to the argument above. The hope with the present analysis with dwarf galaxies dominated by dark matter, is to obtain the distribution of
with different systematic uncertainties, to better constrain the corrections for the unknown
and the stellar mass-to-luminosity ratio
, and to more reliably extract the adiabatic invariant
.
In section 2 we discuss the hydrostatic equations that describe two self-gravitating gases, dark matter and baryons, and their solutions. In section 3 we obtain
from the rotation curves measured by Se-Heon Oh et al. [6], based on the “Local Irregulars That Trace Luminosity Extremes—The HI Nearby Galaxy Survey” (LITTLE THINGS), and additional infrared and visible images. Systematic uncertainties are discussed in section 4, the final adiabatic invariant is obtained in section 5, followed by comments, a discussion on relaxation, and conclusions. Measured and calculated rotation curves are collected in the appendix.
2. Hydrostatic Equations
The hydrostatic equations that describe two self-gravitating gases (dark matter
![]()
Figure 1. Distribution of
, i.e. the adiabatic invariant before the dark matter rotation correction, of 46 spiral galaxies in the SPARC sample [5].
and baryons) are Newton’s equation, and the time-independent limit of the radial component of Jeans Equation, (a moment of the Collisionless Boltzmann Equation, or
in disguise): [4] [7] [8]
(2)
(3)
(4)
We have included the parameters
and
to describe dark matter and baryon rotation, respectively.
and
, where
is the velocity of a test particle in a circular orbit of radius
in the plane of the baryon disk, and
and
are the contributions, from dark matter and baryons, to
. (
is approximately equal to the galaxy “spin parameter” of P.J.E. Peebles:
, where
is angular momentum, and
is energy). Equations (3) and (4) can also be understood as momentum conservation equations, separately for particles with
and
. These equations are approximate for the problem at hand. (We could have considered separate equations for stars and gas. The equation for gas is the Euler Equation, i.e.
is replaced by the sound velocity). For a full discussion, see [9].
We make the data driven approximations that
,
,
and
are independent of
, since, with these approximations, we obtain excellent fits to the galaxy rotation curves, see the appendix, and references [5] and [8]. These approximations can be understood as follows. The (arguable) independence of
and
on
corresponds to thermal equilibrium, i.e. to the non-relativistic Boltzmann distribution, separately for dark matter and baryons, and is non-trivial, see the beautiful discussions in the chapter “The exponential atmosphere” in “The Feynman Lectures on Physics” to appreciate the subtleties of thermal equilibrium, and to understand the right-hand-side of Equations (3) and (4). In the warm dark matter scenario, the radius of the halo grows with velocity
, and the particles becoming bound by gravity populate the tail of the non-relativistic isothermal Boltzmann distribution [10]. Note that if
at large
,
independent of r results in the observed flat rotation velocity
at large
. As we shall see,
, while
is largely unknown.
Note that (3) depends on
, and (4) depends on
, so, to measure
from rotation curves, it is necessary to obtain
from an independent source.
We consider the case
that corresponds to the dwarf galaxies in the present study, and assume spherical symmetry. There is a 2-parameter family of solutions, defined by the core density
and
. Given these boundary conditions, the solution has a core radius
, and an asymptotic density, given by
(5)
The adiabatic invariant (1) in the core of the galaxy is
(6)
The results in (5) can be approximated by the “pseudo-isothermal sphere”
(7)
(the exact solution is given in [9] [11]) corresponding to the circular velocity
(8)
Note that the circular velocity at large
obtains
, while the slope of the circular velocity at small
obtains
. These two parameters obtain
, i.e. the adiabatic invariant uncorrected for dark matter rotation.
The solution for baryons at large
is
with
. At the time of the galaxy formation,
[4]. As baryons loose energy by radiation,
increases, and baryons become localized, i.e. their mass within large
eventually converges, when
.
3. Data Analysis
The data source of the present study is obtained from the Se-Heon Oh et al. state-of-the art analysis in [6], based on the LITTLE THINGS survey [12], combined with Spitzer 3.6 μm images [13], and additional U, B, and V optical images [14], to obtain mass models of 26 late-type dwarf galaxies. The LITTLE THINGS survey collects data with the very large radio interferometer array Karl G. Jansky VLA1 that observes the 21 cm hyperfine line of atomic hydrogen (HI) of nearby dwarf galaxies (at distances less than 11 Mpc, with ≈ 6” angular resolution, and <2.6 km/s velocity resolution [12]).
From the 26 dwarf galaxies studied in [6], we select those that satisfy these criteria: 1)
to obtain a reliable dark matter rotation curve; 2)
reaches
so
is well measured; and 3)
has a rising segment at small
so
is well measured. Finally, we remove the blue compact dwarf galaxy Haro 29 that is extremely irregular [6] (see the rotation curve at the end of the appendix), indicating a probable recent interaction [15]. This selection leaves the 11 galaxies listed in Table 1, at distances between 3 Mpc and 11 Mpc, except WLM at 1 Mpc, i.e. outside of the Milky Way.
The Se-Heon Oh et al. collaboration [6] obtains the galaxy rotation curves, subtracts the baryon (gas and stellar) contribution, fits the resulting dark matter rotation curves to the pseudo-isothermal template (8), and obtains
and
. From
and
, we obtain the adiabatic invariant
of warm dark matter, uncorrected for dark matter rotation, as follows:
(9)
Note that a knowledge of
, independently of the rotation curves, is needed to apply the correction factor
, to finally obtain the adiabatic invariant
. The results are summarized in Table 1 and Figure 2. The mean and standard deviation of
are 0.568 km/s and 0.208 km/s, respectively.
As an alternative analysis, we integrate numerically Equations (2)-(4) in spherical coordinates, starting from the first measured point at
, and ending at the last measured point at
. To start these integrations it is necessary to provide four boundary conditions:
,
,
, and
. These four parameters are varied to minimize a
between the measured and calculated rotation curves. The uncertainties of
are provided in [6]. To define the
it is necessary to assign uncertainties to the baryon contribution. We do this by eye, guided by the fluctuations in the baryon contribution (that is sub-dominant). The data is compared with the numerical integrations in the
![]()
Table 1. Presented are 11 dwarf galaxies passing the selections (see text), their central dark matter density
, and core radius
, from [6], and the calculated
from (9). Uncertainties are statistical from [6]. The mean and standard deviation of
are 0.568 km/s and 0.208 km/s, respectively.
![]()
Figure 2. Distribution of
, i.e. the adiabatic invariant before the dark matter rotation correction, of 11 dwarf galaxies from Table 1 (upper panel), and Table 2 (lower panel).
appendix. The results are summarized in Table 2 and Figure 2. The mean and standard deviation of
are 0.490 km/s and 0.160 km/s, respectively. Note that
depends mainly on the data points of
at large
, while
depends mainly on the data points at small
.
The two distributions in Figure 2, corresponding to Table 1 and Table 2, are different. The difference has contributions from the different assumptions of these two analyses. Table 1 assumes the “pseudo-isothermal sphere”, i.e. fits to the template (8), while Table 2 assumes dark matter is exactly isothermal, i.e.
. The difference between these two assumptions is non-negligible, and is studied in detail in [11]. In the present article we favor
because it corresponds to dark matter particles with the non-relativistic isothermal Boltzmann distribution that is justified in [1].
We choose Table 2 as our final result for the adiabatic invariant before correcting for rotation (which is also the method used in [5]). The distributions in Table 2 and in the SPARC sample [5] are compared in Figure 3.
4. Systematic Uncertainties
If dark matter is cold, the distributions in Figures 1-3 are the result of galaxy formation, relaxation and “virialization”. In this case, what sets the scale of
, and why is its distribution so narrow relative to the mean? We consider the alternative, that dark matter is warm, and
is an adiabatic invariant of cosmological origin. If dark matter is warm, what phenomenon sets the width of
?
We consider these sources of systematic uncertainties:
1) Stellar mass-to-light ratio
. The uncertainty from this source can be estimated by running the fits with several values for
. For the 11 studied dwarf galaxies this uncertainty is negligible, unless
is greater than approximately
. For the set of 11 dwarf galaxies we set
, and ignore this uncertainty.
2) “Phase space dilution” may be an issue if the galaxy formation has relaxation,
![]()
Table 2. Presented are 11 dwarf galaxies passing the selections (see text), their reduced radial velocity dispersions
and
, and their core densities
and
(calculated by integrating numerically the hydrostatic Equations (2)-(4), see text), and
calculated with (6). The comparisons of the observed and calculated rotation curves are presented in the appendix. Uncertainties are statistical at 68% confidence. The mean and standard deviation of
are 0.490 km/s and 0.160 km/s.
![]()
Figure 3. The expansion parameter at which dark matter becomes non-relativistic (uncorrected for dark matter rotation)
, as a function of the absolute luminosity, of the 11 LITTLE THINGS dwarf galaxies in Table 2, and the 46 spiral galaxies in the SPARC sample [5]. The absolute luminosity is in the V-band for the LITTLE THINGS sample, and 3.6 μm for the SPARC sample.
i.e. is not adiabatic. For warm dark matter, first galaxies form adiabatically [4]. In the cold dark matter scenario, galaxy formation requires relaxation and “virialization”. What happens to the adiabatic invariant
when two galaxies collide, relax and merge? Does relaxation “dilute” phase space? The answer is yes and no. According to the Collisionless Boltzmann Equation, the “fine-grained” phase space density, i.e. the number of particles per unit phase space volume, as seen by an arbitrary particle, is constant (in the approximation that the particles are collisionless and conserved). However the “coarse-grained” phase space density can become “mixed with vaccuum”, i.e. “diluted”. Thus the coarse-grained adiabatic invariant
can increase due to relaxation (see the discussion on the coarse-grained entropy in [9], and see section 7 below).
3) Baryon disturbances due to interactions. The adiabatic invariant of a galaxy is obtained from the observed HI rotation curve
, and the observed baryon (gas plus stars) density
. An interaction that disturbs baryons will change the observed adiabatic invariant. From the 11 dwarf galaxies studied in this analysis, about 7 rotation curves show extraneous features, see the figures in the appendix. An extreme example is Haro 29, which appears to have had a recent interaction [15] which has distorted the measured rotation curve, see the last figure in the appendix. For Haro 29 we obtain
km/s (with a large
per degree of freedom), i.e. a fluctuation from the mean 0.490 km/s of 0.287 km/s! For these reasons we estimate that baryon perturbations are a main source of uncertainty of the measured
, and may reasonably reach an uncorrelated ± 0.1 km/s per galaxy.
4) Dark matter halo rotation. One interpretation of the width of the distributions of
is the dark matter halo rotation correction factor
that is different for each galaxy. If the lower edge of these distributions at
km/s correspond to
, then the distribution for the 11 dwarf galaxies has
in the range 0 to approximately 0.8, see Figure 4, while the distribution for the 46 SPARC spiral galaxies has
in the range 0 to approximately 0.95.
Note that the uncertainty of the measured
due to relaxation and rotation is limited to the width of the distributions in Figure 2. Furthermore, both rotation and relaxation increase
, so at the lower end of the distribution of
we should have
. Finally, according to Table 2, rotation and relaxation only increase the adiabatic invariant by a factor at most 2.2.
Without further knowledge of the rotation and relaxation corrections, we can neglect these corrections altogether, and present, as a conservative estimate, the mean and standard deviation of the distribution in Table 2:
(10)
In the following sections we try to improve over this estimate.
5. Interpretation
To obtain the adiabatic invariant
we still need to obtain
for each
![]()
Figure 4. Shown are
vs.
from Table 2, with statistical uncertainties only. The lines correspond to the adiabatic invariant
km/s, and
(red continuous line), or
(blue dashed line).
galaxy. This rotation parameter can not be obtained from the rotation curves, so we need independent data, or assumptions, to proceed. In Figure 4 we present
vs.
for each galaxy in Table 2, indicating statistical uncertainties only. Superimposed on this figure we present lines corresponding to
km/s, for
and
. So, one interpretation is that galaxies near the continuous line have
, while galaxies to the right of this line have
(or some other disturbance).
The distribution of
is peaked at the low end, see Figure 2 and Figure 4. We interpret this peak as corresponding to galaxies with little dark matter rotation, i.e.
, which are therefore likely to have experienced no major mergers. Note that a warm dark matter halo has less small scale structure than a cold dark matter halo, and so less baryon angular momentum is transferred to the warm dark matter [16]. We interpret the width of the
peak as due to baryon disturbances, and residual dark matter halo rotation and relaxation. Since we have no reliable systematic uncertainties, we simply quote the mean and standard deviation of
of the eight dwarf galaxies with least
in Table 2, assuming they have
, see Figure 4:
(11)
The same result is obtained, from these eight dwarf galaxies in the
peak, if we add (in quadrature), to the statistical uncertainties, an uncorrelated systematic uncertainty ± 0.19 km/s. To add the remaining three galaxies would require corrections for rotation and relaxation for these three galaxies.
Similar, complementary and/or differing viewpoints can be found in [17] [18] [19].
6. Comments
In Table 2 we note that
, or
. Is there a reason for this? Dark matter and baryons share the same gravitational potential. For a first generation galaxy we expect that
is of order
, before baryons loose energy by radiation, see simulations in [4]. Note that the numerical solutions in [4] obtain
, or
, before baryons loose energy by radiation. The relation
is also approximately valid for the sample of dwarf galaxies under study, see the figures in the appendix. So, from the virial theorem applied to a sphere of radius
, separately for dark matter and baryons, we can expect that the kinetic energy per unit mass is similar for dark matter and baryons. Let
be the mean kinetic energy of a dark matter particle in the sphere of radius R. Then, twice the dark matter mean kinetic energy per unit mass, assuming equal dispersions in the 3 dimensions, is
(12)
A similar equation holds for baryons. If dark matter and baryon particles are mainly supported by rotation, i.e. if
and
are approximately 0.99, then
. If dark matter is supported mainly by dispersion, i.e.
, while baryons are supported mainly by rotation, then
. In conclusion, the approximate equality of
and
can be understood.
The observed baryon 1-dimensional velocity dispersion, in the sample of 11 dwarf galaxies, e.g. DDO 43, is
km/s [6]. For
km/s, we obtain
. If
, then
, so
, and dark matter particles rotate on very nearly circular orbits. If
, then
, so
, and baryons rotate on nearly circular orbits, in a plane due to collisions, as observed. In the warm dark matter scenario, velocities in first generation galaxies are nearly isotropic in the core, and increasingly radial at larger r (from the simulations in [4]), while collisions and mergers may change this.
7. Relaxation
The following thought toy experiments have helped me try to understand relaxation. Consider warm dark matter in a box. The dark matter particles are collisionless, except with the walls of the box (that represent gravity). Now put two boxes side-by-side, one full and one empty of dark matter, and remove the dividing wall. Particle velocities are unchanged. The particle density is halved, so the “coarse-grained” adiabatic invariant has increased by a factor 21/3. If velocities are reversed exactly, the dark matter particles return to their original box, so the “fine-grained” adiabatic invariant has remained unchanged.
Now consider two full boxes that collide. At the moment of the collision the boxes are stopped, and the dividing wall is removed. The dark matter density is unchanged. The dark matter particle velocities are unchanged. However, the velocity dispersion with respect to the center-of-mass has increased, so the “coarse-grained” adiabatic invariant has increased. If the collision is offset, the dark matter acquires angular momentum, which again increases the observed
.
We can now understand the three galaxies farthest to the right of the continuous red line in Figure 4: these galaxies have undergone one or more major interactions and mergers, and so have acquired angular momentum and/or relaxation, resulting in an increase of
. On the other hand, the eight galaxies near the continuous red line are pristine (in our interpretation), they have little rotation, i.e.
, and have not encountered major interactions. These pristine dwarf galaxies should contain a few first or second generation stars with little or no metallicity.
8. Conclusions
The results summarized in Table 2 are obtained directly from the rotation curves in reference [6] of 11 dwarf galaxies dominated by dark matter, see the excellent fits in the appendix. The distribution of
in Table 2 and Figure 2 has a mean 0.49 km/s, a standard deviation 0.16 km/s, and is peaked at low
. We apply a mild, data driven, rotation and relaxation correction that obtains the adiabatic invariant in the core of the galaxies:
(13)
These two small relative standard deviations justify the prediction that the adiabatic invariant
in the core of the galaxies is of cosmological origin if dark matter is warm. This result is in agreement with independent measurements of
, summarized in Table 3 of [20], based on spiral galaxy rotation curves, galaxy rest frame ultra-violet luminosity distributions, galaxy stellar mass distributions, the formation of first galaxies, reionization, and the velocity dispersion cut-off mass.
Appendix. Circular Velocities and Densities
The figures below present the circular velocities
, and densities of dark matter
and baryons (gas plus stars)
, and their sum
, as a function of the distance
from the center of each galaxy.
is the velocity of a test particle in a circular orbit of radius
in the plane of the baryon disk, and has contributions from dark matter and baryons:
. The data points are obtained from [6]. The continuous lines are obtained by numerical integration of the hydrostatic equations (2), (3) and (4), that require four boundary conditions to start the integration [8]:
,
,
, and
, where
is the smallest measured
. These four parameters are varied to minimize a
between the data and the numerical integration. The results are presented in Table 2. The indicated uncertainties of
are estimated from their fluctuations.
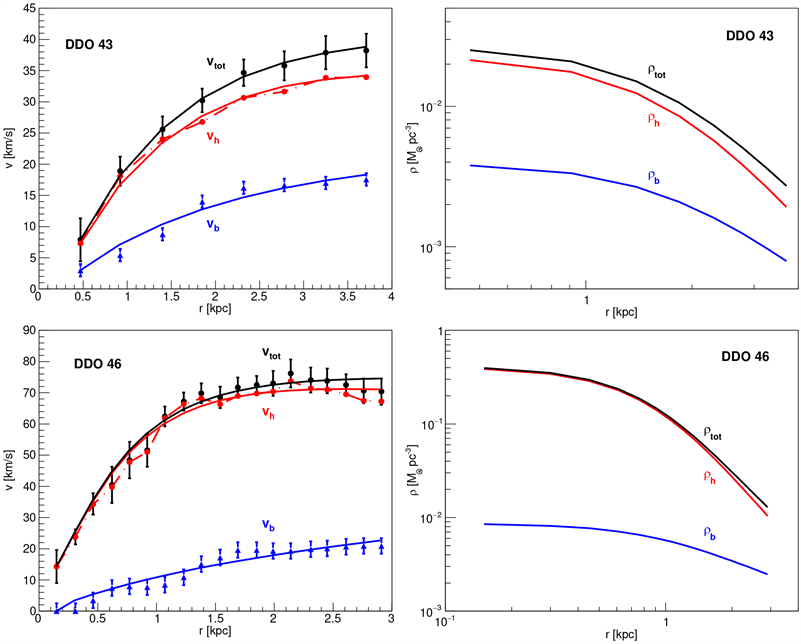
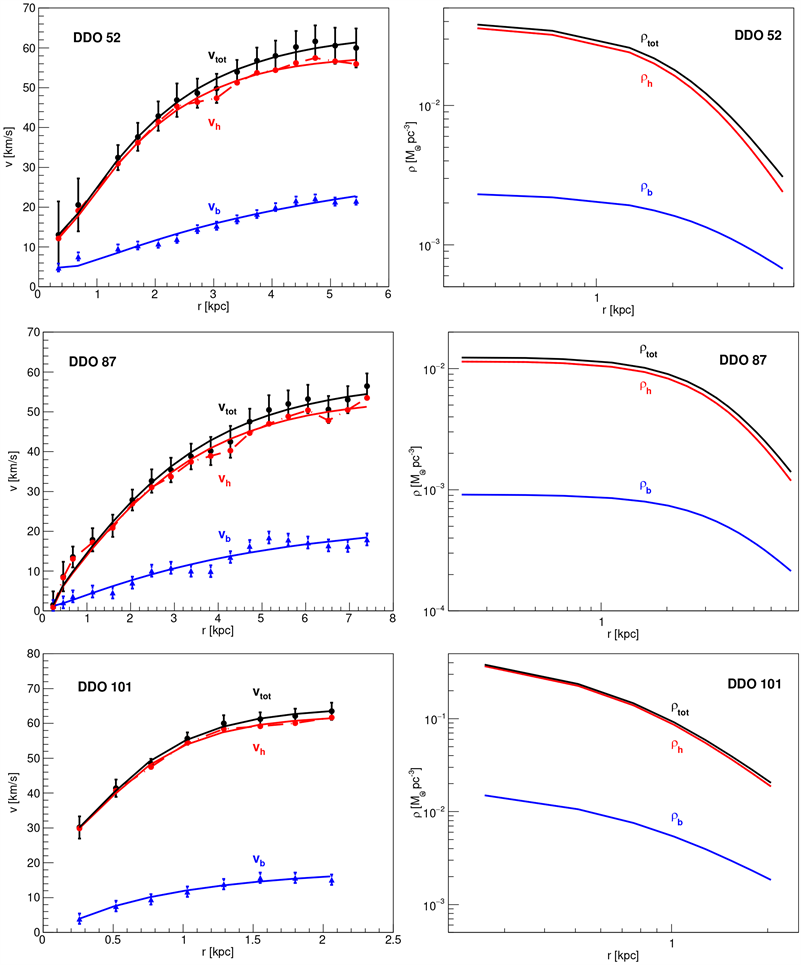
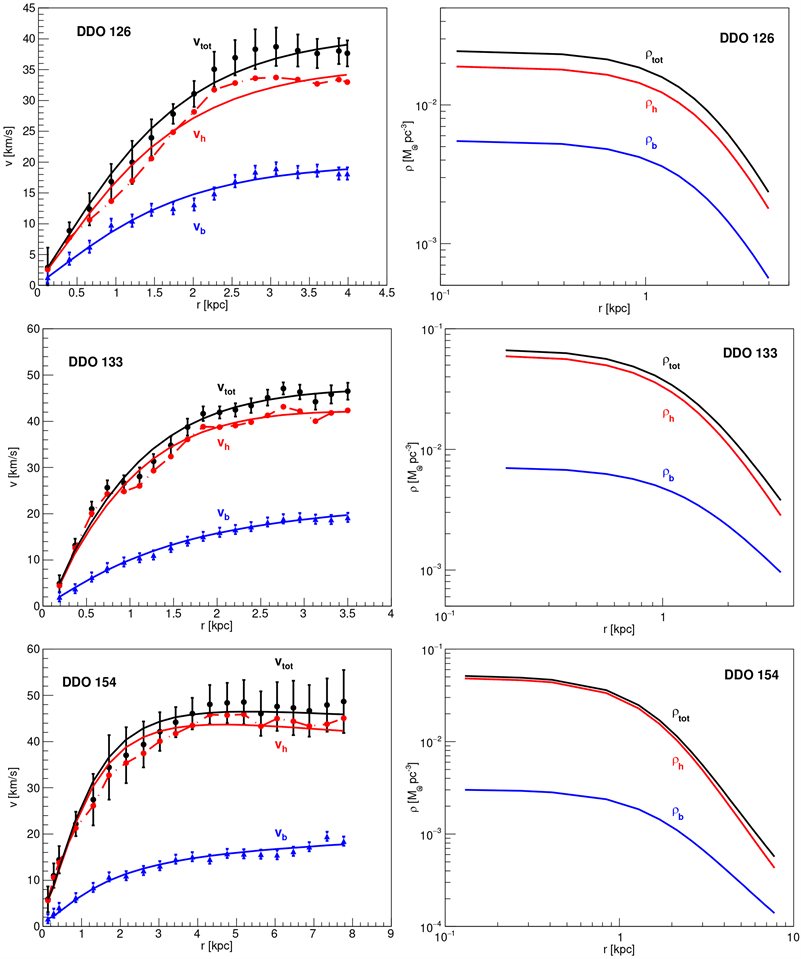
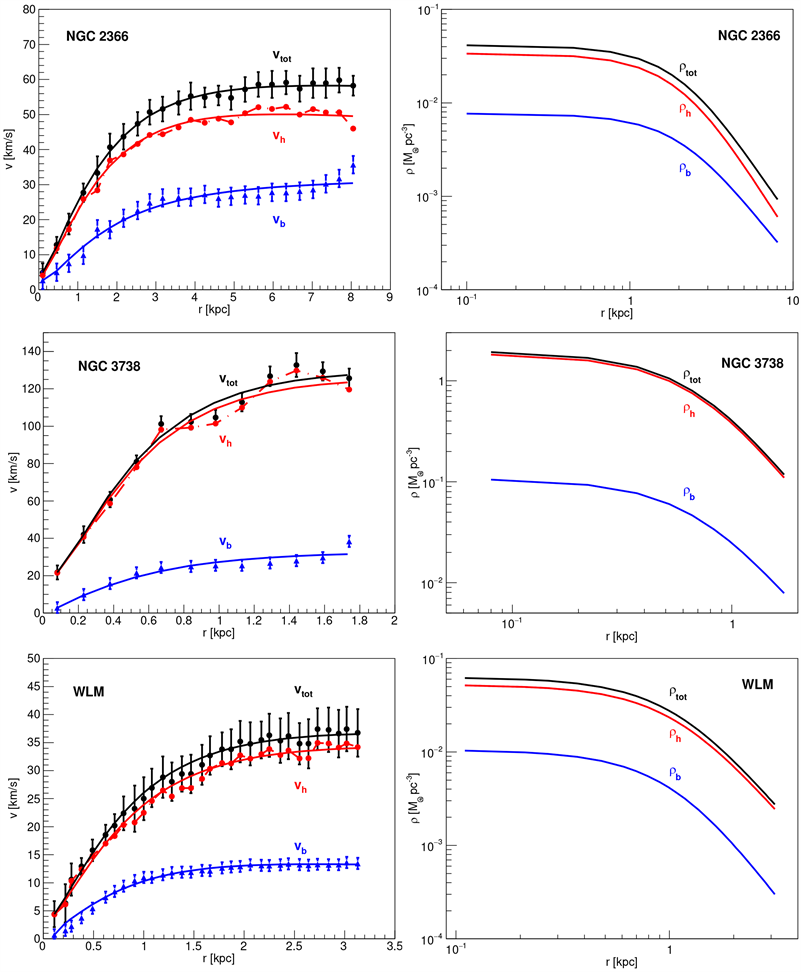
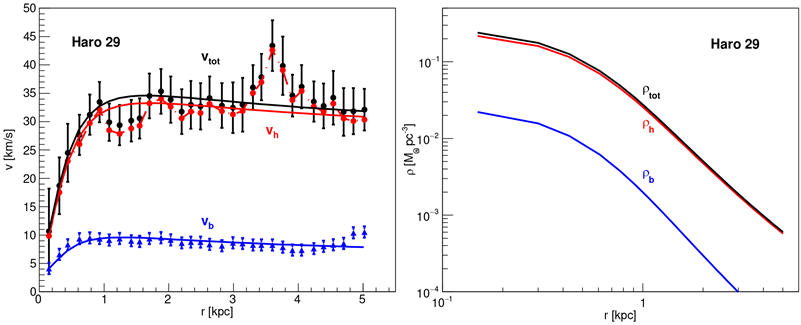
NOTES
1The VLA is a facility of the National Radio Astronomy Observatory (NRAO). The NRAO is a facility of the National Science Foundation operated under cooperative agreement by Associated Universities, Inc.