Review: Neuronal Differentiation Protocols of Mesenchymal Stem Cells ()
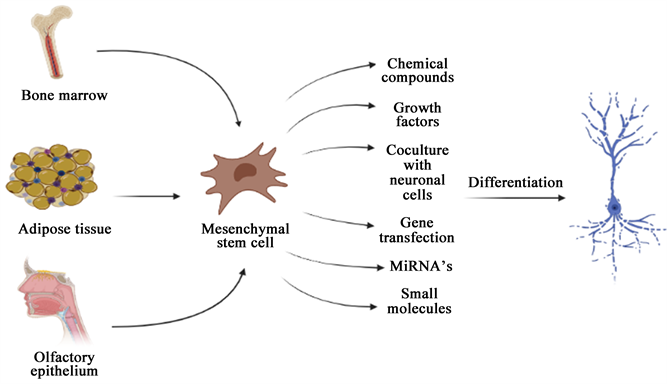
1. Introduction
Mesenchymal stem cells (MSC), also known as multipotent mesenchymal stromal cells, are present in almost all postnatal organs and tissues, particularly in the perivascular region [1]. These cells are characterized by a spindle-shaped morphology with an elongated central nucleus and contain 2 to 3 nucleolus. Three subpopulations of these cells have been described by flow cytometry: 1) small fusiform and granular cells, 2) small agranular cells, and 3) large granular cells. By microscopy, two morphological types have been reported in culture, a fibroblastic shape (predominant) and more giant cells with rhomboid shape [2].
According to the International Society of Cell Therapy’s Committee on Mesenchymal and Tissue Stem Cells, MSCs must meet three minimum criteria: 1) they must be adherent to plastic under standard growing conditions; 2) must express the CD105, CD73, and CD90 markers (more than 95% of the cell culture), as measured by flow cytometry, and should lack the expression (less than 5%) of CD45, CD34, CD14, CD11b, CD79a, CD19, and HLA Class II; and 3) finally, the cells must have the ability to differentiate into osteoblasts, adipocytes, and chondrocytes, using standard conditions for in vitro culture differentiation (Figure 1). Differentiation to osteoblasts can be demonstrated by red alizarin or von Kossa staining; Oil Red staining quickly demonstrates adipocyte differentiation and chondrocyte differentiation, shown by Alcian blue 8G and collagen type II [3].
In theory, mesothermal differentiation is easily achievable for MSC because they have the exact embryonic origin. However, several studies have provided evidence that under optimal in vitro growing conditions or in vivo cardiac niches, MSC may also result in other types of highly specialized tissues, including cardiomyocytes and endothelial cells [4] or endodermal lineages, such as hepatocytes [5] and pancreatic beta cells [6]. In addition, numerous studies have
![]()
Figure 1. According to the International Society of Cell Therapy’s Committee on Mesenchymal and Tissue Stem Cells, minimum criteria to be considered mesenchymal stem cells.
shown that these cells also can differentiate into ectodermal lineages such as nerve tissue [7] [8] [9].
In vitro reprogramming of MSCs towards the neuronal lineage can be carried out using different methodologies with different inducers, such as chemical substances, growth factors, co-cultivation with neural lineage cells, gene transfection miRNA, and chemicals called small molecules. These methodologies show considerable variations in terms of efficiency of differentiation. The choice of the various differentiation inducers depends on several factors, such as the price and the intrinsic characteristics of the cells used to induce the differentiation process. It will depend on the presence or absence of the receptors required by these inducers and on the expression of the molecules involved in the differentiation pathways that the different inducers must specifically activate. Therefore, this article aims to compare multiple experimental tests in which these inducers promote the neuronal differentiation of MSCs. The analysis will identify an optimal neuronal differentiation method with reasonable differentiation rate, maturation, expression of neuronal markers, functionality, and cell survival considering the intrinsic characteristics of the MSCs used as the tissue of origin and the species from which they were isolated.
2. MSC History
In 1999 Pittenger et al. characterized an isolated population of homogeneous human mesenchymatous cells derived from bone marrow, highly proliferative in culture, with an extended fibroblastic morphology. These cells showed a constant set of surface proteins expression and high differentiation capacity towards adipocytic, chondrocyte, and osteocytic lineages. All these characteristics were maintained in cell colonies derived from individual cells (clones), avoiding the possibility that the bone marrow contained only progenitor cells committed to the hematopoietic lineage [10].
In addition to bone marrow aspiration, MSC has been isolated from a variety of other adult tissues, such as adipose tissue aspirates and other connective tissues, such as the medullary space of long bones, fragments of trabecular bone, periosteum, synovial fluid, periodontal ligament, palatine amygdala, parathyroid gland, and fallopian tube, pancreas, liver, dermis, and adult skeletal muscle [2] [11]. Similarly, MSC can be extracted from other tissues such as Wharton’s jelly umbilical cord, temporary tooth pulp, amniotic fluid, amnios, and placenta [11] [12] (Figure 2).
Recently, a population of stem cells with mesenchymal characteristics was isolated from the nasal epithelium. These cells have a cell phenotype characterized by the expression of surface proteins common to MSC, including STRO-1, CD90, CD105, and Nestine, an intermediate filament. They do not express markers typical of the olfactory epithelium, such as cytokeratin’s, nor hematopoietic lineages, such as CD34 and CD45. It is important to note that these cells are obtained from lamina propia and differ markedly from the basal cells of the olfactory epithelium, which act as stem cells for the neuroepithelial lineages [13].
Nasal MSC is transcriptionally more related to bone marrow MSC and has shown significant differences with other stem cells such as hematopoietic stem cells, neural progenitor cells, synovial fibroblasts, and periosteum cells. Additionally, nasal MSC shows a high proliferation rate compared to bone marrow MSC, differentiating into bone cells and neurons, and has little differentiation capacity to chondrocytes and adipocytes since chondrogenesis inhibitors (Asporin) adipogenesis (GATA2) are positively regulated. At the same time, neurogenesis (Neurogenesis-1) and osteogenesis (OSR2) activators are overexpressed in these cells. In addition, since nasal MSC originates from tissue derived from the neural crest, they exhibit more significant expression of genes related to neural cells, so they have been referred to as ecto-mesenchymal stem cells (eMSC) [14].
Cultured cells must present similar criteria to be considered as MSC, regardless of the site from which they are obtained; however, some differences have been found regarding the proliferative capacity and expandability among some MSC depending on their origin, for example, bone marrow and adipose tissue MSC have greater culture efficacy than umbilical cord MSC, but the latter has more significant expansion potential compared to other cell sources [15].
3. Multipotency of MSC
The multipotential capability of MSCs, are related to the expression of specific molecules of the mesenchymatous lineage such as vimentin and fibronectin, collagen type I and III, specific factor 2 of osteoblasts, and type VI collagen, and they also express mRNAs common for different kinds of other cell lineages, including osteoblasts, chondrocytes, and myoblasts. In addition to this, the expression of atypical molecules of the mesenchymal cells themselves has also been shown, such as mRNAs characteristic of epithelial and endothelial cells, such as cytokeratin (CK) 8 and 10, vascular endothelial growth factor (EVGF), and collagen IV, among others. MSCs also express transcripts common to neural tissues, such as Neurofilament H, high-affinity nerve growth factor receptor (NGFhi), Nexine 1 alpha, derived from glia, subunit 2C of N-methyl-D-aspartate glutamate receptor, as well as various neurotrophic factors [16]. On the other hand, MSC obtained from fetal tissues has been observed to express genes related to embryonic stem cells, such as the leukemia inhibitor factor receptor (LIFR), ESG1, SOX-2, Oct4, FGFR4, Nanog, Rex1, and TERT, as well as genes for proteins associated with morphogenesis such as SHH, Neuregulin1 and 4, Patched, SNA2 and WNT4 [17].
4. Function and Properties of MSC
MSCs have remarkable plasticity, allowing them to differentiate to different lineages under the appropriate stimuli. It has been proposed that these cells could function as natural tissue restorers and therefore can be used to restore various cell populations after transplantation. Not only their differentiation potential makes them an ideal candidate for this purpose, but several trophic properties that promote regeneration in the surrounding tissue, including 1) secretion action [17] - [22], 2) migration capacity [23] - [33] and 3) immunomodulatory action [12] [34].
5. Competitive Advantages of MSC
Several unique characteristics of adult mesenchymal stem/progenitor cells are essential for therapy. MSC can be stored for long periods without a significant loss of their properties or potency. In addition, it can be collected from a variety of adult tissue, unlike embryonic stem cells, whose use is not aggravated by ethical considerations or legal restrictions. [11]. MSC possesses immune privilege and immunomodulation at every point of the cell cycle. It is propagated to produce the tissue of interest [35] and, most importantly, does not present tumorigenic potential [36]. Likewise, MSC can modulate the immune response since allogeneic transplantation of these cells occurs without a substantial risk of immune rejection, even in the absence of immunosuppressive agents [25]. In addition, MSC has excellent stability after storage, freezing, and thawing do not affect their stemness, nor their cellular properties and functions. And do not induce changes in surface protein expression, activated gene profile, and differentiation or proliferation capacity [37].
A decisive role in MSCs characteristics is the age and health of the source of these cells. It has been observed that stromal bone marrow cells isolated from healthy individuals under 50 years of age have a higher osteogenic differentiation capacity than cells obtained from individuals over 60 [38]. In addition, for health status, it has been observed that MSC obtained from people with obesity has a significantly deteriorated proliferation and differentiation capacity [39].
6. MSC and Nervous System Diseases
Some areas of the brain can grow or deteriorate depending on the cognitive demands from the environment, and acute aggressions can stimulate adult neurogenesis. However, supported by neural stem cell niches, resident progenitor neurons do not compensate entirely for the harmful consequences of severe trauma or neurodegenerative diseases. Therefore, stem cell culture could provide an exogenous cell therapy and be proposed as an attractive alternative to treat various neurological disorders. Several stems or progenitor cells have been proposed to treat brain injuries, such as neural progenitors and embryonic stem cells. However, ethical and technical issues associated with these cells have driven new strategies based on autologous grafting of adult mesenchymal stem cells [30]. MSC can be differentiated into different lineages under the appropriate induction conditions. Therefore, it has been proposed that these cells could restore damaged cell populations after transplantation in nervous system diseases. In fact, not only their differentiation potential makes them an ideal candidate for cell therapy, but the secretion of micro vesicles (exosomes) that promote regeneration in the surrounding tissue is crucial [32].
MSCs must fulfill the following criteria for Cell Therapy Treatment in degenerative neuron diseases: 1) donor cells should be readily available; 2) must be highly proliferative with rapid expansion capacity in cell culture; 3) should be immunologically inactive; 4) must survive for a long time and integrate into the host’s brain; 5) and should be able to maintain a stable transfection and long-term expression of exogenous genes if that is required for the therapy [27].
MSC has all these characteristics and have become excellent candidates to be applied in cell therapy protocols, demonstrating their usefulness in functional recovery in various models of neurodegenerative diseases and nervous system injuries, like ischemic stroke [18] [30] [40] [41] [42] [43], demyelinating injury [44] [45] [46], spinal cord injury spinal [20] [47] [48] [49], paraplegia [50], Parkinson’s disease [17] [51] and cochlear injury [13].
Despite the positive results obtained in these trials using undifferentiated MSCs, some studies suggest that the therapeutic effects obtained by MSC transplantation are potentiated when using differentiated MSCs towards the neuronal lineage before cell transplantation [52] [53]. In this sense, several methods induce in vitro reprogramming of MSCs towards the neuronal lineage. The use of chemical substances, growth factors, co-cultures with neural lineage cells, gene transfection,
![]()
Figure 3. Neuronal differentiation methods for MSCs.
miRNAs, and small molecules (Figure 3).
7. Chemical Compounds
Differentiation through chemical compounds has been one of the most used protocols to promote a rapid differentiation of MSCs towards a neural phenotype; within these substances, the use of antioxidants stands out, drugs that increase the levels of cyclic adenosine monophosphate (cAMP) and modifiers chromatin as inducers of transdifferentiation.
7.1. Antioxidants
7.1.1. β-Mercaptoethanol
Woodbury et al. were the first to use β-Mercaptoethanol (β-Me) as an inducer in differentiating murine bone marrow MSCs into a neuronal phenotype. For this purpose, the MSCs are exposed to a pre-induction medium with β-Me at low concentrations for 24 hours to replace this medium later utilizing neuronal induction with a higher chemical concentration. This method promotes that as early as 3 hours after culture in induction medium, the MSCs progressively assume neuronal morphological characteristics. Initially, the cytoplasm of the cells retracts towards the nucleus, forming a contracted multipolar cell body, leaving membranous extensions in the form of peripheral processes. The cell bodies become increasingly spherical and refractile two hours later, exhibiting a typical neuronal appearance, showing primary and secondary branches, growth cone-shaped terminal expansions, and putative filopodial extensions. Furthermore, this morphological change is accompanied by an increase in the neuronal marker neuron-specific enolase (NSE) expression in the treated MSCs, compared with control MSCs [54].
In this regard, Zuk et al. treated human MSCs derived from adipose tissue with this protocol, which produced that just 30 minutes after induction, a change in the morphology of MSCs was observed, in which around 10% of the cells assume a neuronal phenotype, which it increases to a maximum of 70% at 3 hours post-induction. This induction results in the expression of neuronal markers NSE and neuronal nuclear antigen (NeuN) in treated MSCs, the expression of which is not detected in MSCs before treatment. However, the MSCs induced by this protocol do not express mature neuronal markers such as microtubule-associated protein 2 (MAP-2) or neurofilament 70 (NF-70), nor do they express glial markers such as galactosyl ceramidase (GalC) or acidic fibrillar protein of the glia. (GFAP) [55].
This protocol has also been used successfully to differentiate human MSCs derived from bone marrow, finding that 5 hours after adding the antioxidant, changes are observed in the morphology of these cells towards a neuronal morphology. However, after a while, the morphology of these cells returns to its basal condition. This treatment also induces the de novo expression of NF-H in the treated cells and an increase in NSE and NeuN, expressed at deficient levels in control MSCs. Immunohistochemical analysis shows that up to 98% of the cells stain with antibodies directed against the early neuronal markers NeuN and β-tubulin III. At the same time, no expression of MAP2, GFAP, or Gal-C is observed, as reported by Zuk and collaborators. The electrophysiological analysis shows the presence of a sodium current (Na+), which is inactivated very quickly, and an output current, probably of K+ in induced MSCs. Similarly, these cells experience an increase in the intracellular concentration of Ca2+ when challenged with a high concentration of glutamate or potassium (K+). At the same time, in undifferentiated MSCs, no significant change is observed in the concentration of this ion [56].
For their part, Khanabdali et al. also reported increases in the expression levels of Nestin, MAP2, and Tau in murine bone marrow MSCs treated with β-Me, compared to the control group, which expresses these markers at deficient levels. At the same time, positive staining of these cells is observed for Nestin and β-tubulin III [57].
Finally, Shi et al. demonstrated that using a lower concentration of β-Me in the induction step does not affect cell differentiation. As early as 5 hours after induction, 70% of murine bone marrow MSCs treated with half of the concentration of β-Me used in the previous tests show characteristic morphological changes of neuronal cells. Likewise, an increase in the expression of NSE and Nestin is observed in the induced cells, while the expression of Notch 1 and the downstream Notch gene, Hes1, are reduced after induction. On the other hand, when treating the cells with hydrogen peroxide (H2O2), Notch 1 and Hes1 increase, suggesting that the expression levels of these molecules correlate with changes in the level of reactive oxygen species (ROS), mediated by treatment with β-Me [7].
7.1.2. BHA/DMSO
Woodbury et al. tested the effect of treating pre-induced cells with β-Me, adding dimethyl sulfoxide (DMSO) and butylated hydroxyanisole (BHA) in the induction step. This modification induces neuronal morphological changes with a time course similar to that of β-Me, where the majority of cells that show rounded cell bodies express high levels of NF-M, tau, and NeuN after exposure to these antioxidants, flat, undifferentiated cells do not. To optimize the differentiation protocol further, the authors replaced the β-Me in the induction medium with basic fibroblast growth factor (bFGF) and then induced MSCs with DMSO/BHA. It promotes an increase in the proportion of cells with neuronal characteristics since most MSCs treated in this way exhibit neuronal morphologies. Approximately 78% of the cells stain positively for NSE and 79% for NF-M [54].
Hou et al. also used DMSO/BHA as inducers to differentiate human MSCs from umbilical cord pretreated with β-Me. After induction, 70% of the cells exhibit the neuron-like phenotype, and 71.4% of all induced MSCs are NSE positive, and 73.2% for NF. Furthermore, toluidine blue staining indicates the presence of Nissl bodies in the cytoplasm of cells, characteristic of neurons. However, the electrophysiological analysis of these cells does not show the presence of the typical Na+ current of the neuron, which suggests differentiation towards an immature neuronal state [58].
For their part, Vaquero et al. studied the effect of adding cerebrospinal fluid before subjecting bone marrow human MSCs to treatment with β-Me, DMSO, and BHA. The addition of cerebrospinal fluid to the culture medium does not significantly differ in the percentage of cells showing neural differentiation markers. One week after induction, the rate of cells that express neuronal markers is 92% for NSE, 90% for NF-200, and 86% for β-tubulin III when previously treated with cerebrospinal fluid, versus 90%, 86%, and 90%, respectively, when omitting the addition of this [59].
7.1.3. Tetramethyl Pyrazine
Nan et al. treated umbilical cord-derived human MSCs with tetramethyl pyrazine, a separate and purified active alkaloid from the Chinese plant Ligusticum wallichii, exhibiting antioxidant properties. After treatment with tetramethyl pyrazine for 30 minutes, 40% of the cells show neuronal morphology, reaching 85% after 90 minutes of treatment. RT-PCR analysis shows that these induced cells show NF-H and NSE expression compared to untreated cells. On the other hand, the results of the immunocytochemical staining show that 80.79% of these cells are positive for NF-H, and 79.76% are positive for NSE [60] (Supplementary TableS1).
7.2. Chemicals That Increase cAMP Levels
Deng et al. treated human bone marrow MSCs with isobutyl methylxanthine (IBMX) and cyclic dibutyryl AMP (dbAMPc), two drugs that increase cAMP levels to induce neuronal differentiation. After six days of induction, approximately 25% of MSCs differentiate into cells with typical neural morphology and increase the expression levels of NSE and vimentin compared to undifferentiated MSCs. However, no changes were observed in the expression level of MAP1B or β-tubulin III between the two populations. Likewise, after removing the inducers from the culture medium, all neuron-like cells die in the following days [61].
Ashjian et al. induced the neuronal differentiation of CMM when culturing them in an induction medium composed of DMEM medium supplemented with insulin, indomethacin, and IBMX. At 14 days of induction, approximately 25% of the total MSC population presents a neuronal phenotype with increased expression of NSE, vimentin, Trk-A, and NeuN, which are expressed at low levels in undifferentiated MSC. Furthermore, after 14 days of differentiation, cells expressing neuron-like morphological characteristics show a late rectifying K+ current. However, these cells do not have GFAP or MAP2 and tau [62].
Long et al. evaluated the neuronal differentiation capacity of human bone marrow MSCs by exposing them to dbAMPc and IBMX and growth factors such as epidermal growth factor (EGF), fibroblast growth factor 8 (FGF-8), and brain-derived neurotrophic factor (BDNF). One day after being cultured under these conditions, approximately 30% of MSCs show neural cell morphology, reaching a value of 70% by day 6. On the other hand, the immunohistochemical assay shows that differentiated MSCs stain positively for the markers β-tubulin III, Nestin, NeuN, NF, gamma-aminobutyric acid (GABA), Tau, and Nurr1, where approximately 90% of these cells express TUJ1 and Nestin at day 14 of induction. In addition, positivity for GFAP and GalC is observed in some cells with glial morphology [63].
Tio et al. studied the neuronal differentiation capacity of human MSCs obtained from the umbilical cord through treatment with a cytokine induction medium of DMEM with low glucose content. They were supplemented with fetal bovine serum (FBS), IBMX, retinoic acid RA, dbAMPc, nerve growth factor-beta (NGF-β), and bFGF. The addition of the medium generates the appearance of neural-type cells in a percentage of 15%. There is an upregulation in the expression levels of Nurr-1, tyrosine hydroxylase (TH), NSE, NF-H, NF-M, NF-L, and GFAP, compared to undifferentiated MSCs. On the contrary, the expression of dopamine beta-hydroxylase is negatively regulated during differentiation. Immunohistochemistry shows high levels of synaptophysin and phosphorylated TH in differentiated cells. Removal of IBMX and dbAMPc from the medium results in fewer neural-like cells. The individual addition of these components to the growth medium results in the generation of some neuron-like cells [64].
Benítez-King et al. Differentiated human MSCs derived from the olfactory epithelium by culturing them in DMEM/F2 medium supplemented with DbAMPc for 24 h. These molecules induced a decrease in the total number of cells and an increased number of cells carrying bipolar and multipolar neurites. Together, the amount of cells stained with the olfactory neuron-specific anti-OMP antibody increases ten times in the presence of DbAMPc, which exhibits a bipolar morphology, and their soma presents a rounded or ellipsoidal shape, compared to control MSCs [65].
In 2016, Shahbazi et al. Evaluated the neuronal differentiation potential of human umbilical cord CMM in the presence of forskolin, another drug that increases levels of cAMP and IBMX. After 8 hours of treatment, morphological changes begin to be observed in some MSCs towards a neural phenotype, which rises 24 h after the induction process. The immunohistochemical assay and western blot result reveal that Nestin, β-tubulin III, NSE, and GFAP are positive after eight hours of treatment in the treated cells. In comparison, the expression of MAP2 is appreciated 24 h post-induction. In contrast, none of these markers are observed in control MSCs. In addition, the combination of forskolin/IBMX improves the phosphorylation of CREB in Ser133, a downstream effector of PKA signaling activated by cAMP, which supports the action of high levels of cAMP in the neuronal differentiation of MSCs [66].
Recently Ayala-Grosso et al. produced neurospheres from human MSCs of the olfactory epithelium by culturing the cells in serum-free DMEM-F12 medium, supplemented with EGF and b-FGF. Subsequently, these mesenchymal neurospheres dissociate into individual cells to differentiate into neuronal lineages on poly-L-lysine-coated coverslips by treatment with RA and forskolin, which generates cells that express the markers β-tubulin III and GFAP [8] (Supplementary TableS2).
8. Chromatin Modifiers
Alexanian et al. treated pre-induced murine bone marrow MSCs with bFGF and EGF, with the hypomethylating agent 5-aza-20-deoxycytidine (5azadC) and the histone deacetylase inhibitor Trichostatin A (TSA) to promote neural induction of these cells. Subsequently, the differentiated cells were cultured in a neural induction medium with or without fixed neuronal stem cells. MSCs treated with epigenetic modifiers exhibit high Sox2 expression compared to untreated cells. In addition, culturing MSCs with neuronal stem cells produces groups or structures similar to neurospheres positive for Sox2 and Nestin, which are enhanced by pretreating the cells with chromatin modifiers. Finally, the results show that MSCs that are preliminarily treated with 5azadC and TSA produces immunopositive cells for β-tubulin III and GFAP with certain similarity to neuronal and glial morphology when maintained in a neurobasal environment, where approximately 70% of the cells show a neuronal morphology [67].
In 2010, this working group induced neuronal differentiation of human bone marrow MSCs by simultaneously exposing cells to DNA methylation inhibitors (RG-108), histone deacetylation inhibitors (TSA). These pharmacological agents increase cAMP (8-BrAMPc and rolipram) and bFGF. Real-time RT-PCR studies show that 24 h after treatment, the expression level of genes associated with Oct 4 pluripotency, Nanog, Klf4, c Myc, and Sox 2 increase in treated MSCs Likewise, the expression levels of the neural genes Nestin, A2B5, NCAM, GFAP, NeuN, MAP2, Nurr1, and TH gradually increase in these cells during the differentiation process. Likewise, these cells can release glial-derived neurotropic factor (GDNF), BDNF, and dopamine to the medium after induction [68].
Two years later, this working group demonstrated that human bone marrow MSCs subjected to hypoxic preconditioning and differentiated by the previous protocol show an improvement in their dopaminergic differentiation since the expression levels of Nurr1 and TH increase significantly in induced MSCs. They were grown under hypoxic conditions, while its counterpart grew under normal oxygen conditions. Likewise, the cell proliferation assay results show that hypoxic preconditioning improves the proliferation and viability of MSCs by protecting them from the cytotoxic effect of H2O2. On the other hand, ELISA studies show that the release of BDNF, GDNF, and dopamine in the treated MSCs is enhanced by cultivating the cells in conditions with low oxygen levels [69].
Zheng et al. compared the differentiation potential of murine MSCs from bone marrow and adipose tissue under these differentiation conditions. MSCs from adipose tissue exhibit a greater proliferation capacity than bone marrow MSCs in vitro. After a seven-day neuronal induction period of 73.61% of MSCs derived from bone marrow present a neuronal morphology cell, while using adipose MSCs, 93.01% of these cells were observed. Furthermore, after treatment, more cells positive for β-tubulin III and ChAT is found in the culture of treated MSCs derived from adipose tissue, relative to bone marrow MSCs. However, a more significant number of MAP2 positive cells is observed when using bone marrow MSCs. In addition, the RT-PCR and ELISA results show a greater expression and release of BDNF but less of NGF and neurotrophin 3 (NT-3) in differentiated bone marrow MSCs compared to those of adipose origin [70].
For their part, Nivet et al. Treated adipose tissue human MSCs with 5-azacytidine, NGF, BDNF, and bFGF to induce their neuronal differentiation. After induction, MSCs show changes in cell morphology, which persist for up to 2 weeks in a neurobasal environment. Specifically, the cytoplasm of some cells retracts toward the nucleus, forming contracted cell bodies with extended cytoplasmic extensions. Increases in Nestin expression accompany these morphological changes and de novo expression of MAP2 and NSE compared to control MSCs. On the other hand, the quantitative analysis shows that one week after neuronal induction, 85.6% of the treated MSCs stain positively for GFAP, while 81.2% do so for MAP2 [30].
Recently in 2017, Fila-Danilow et al., Analyzed the role of histone deacetylase inhibitors TSA and valproic acid in the neuronal differentiation of murine MSCs pretreated with bFGF and EGF. They find that the expression of β-tubulin III and Gal-C increases after three days of treatment in MSCs but decreases after six days. On the contrary, the expression of TH and VAChT increases gradually in these cells during the differentiation process [71] (Supplementary TableS3).
9. Growth Factors
Goodwin et al. treated human MSCs of the umbilical cord and bone marrow in a medium added with bFGF and EGF for seven days for their neuronal differentiation. After treatment, both the umbilical cord and bone marrow MSCs morphologically adopt some of the characteristics of neural cells in culture, such as long bipolar extensions and branched ends. Furthermore, both cell populations express β-tubulin III and GFAP after induction. However, umbilical cord MSCs express the markers only after the differentiation process, whereas some bone marrow MSCs already express these markers constitutively in the standard growth medium but increase their expression with the addition of the growth factors [72].
Dezawa et al. used a combination of growth factors to differentiate murine bone marrow MSCs, for which they first pre-induced these cells by treating them with β-Me and RA for three days. Later they were cultured in an alpha-MEM induction medium supplemented with FBS, Forskolin, bFGF, platelet-derived growth factor (PDGF), and recombinant human Heregulin beta1 (HRG) for seven days. Phase-contrast microscopy reveals that MSCs adopt a morphology similar to Schwann cells after differentiation, unlike control MSCs. Immunohistochemistry shows that most differentiated MSCs, but not the undifferentiated ones, are positive for the Schwann cell markers p75, S100, GFAP, and O4. On the contrary, the omission of β-Me, RA, forskolin, or HRG in the differentiation protocol results in incomplete differentiation of MSCs in both morphology and immunoreactivity [52].
Peng et al. used this same protocol to induce neural differentiation of human MSCs derived from Wharton’s jelly. After induction, these cells change from a flat shape to a bipolar spindle morphology and express Schwann cell markers. Cell quantification shows that approximately 68% of the differentiated cells are positive for GFAP, 77% for p75, 75% for S100, and 72% for MBP. Furthermore, the enzyme-linked immunosorbent assay and RT-PCR show that differentiated MSCs express and secrete BDNF, NGF, and NT-3 into the medium [73].
For their part, Jiang et al. cultured bone marrow murine MSCs in wells coated with fibronectin and bFGF to induce their neuronal differentiation. After 14 days of induction, MSCs acquire morphological and phenotypic characteristics of neuronal and glial cells, defined by their positivity for NF200 (68.9%), GFAP (15.4%), and Gal-C (12.3%). Following bFGF culture, the messenger RNA levels of Otx2, Otx1, Pax2, Pax5, Nestin, MAP2, and Tau are significantly increased compared to undifferentiated MSCs. On the other hand, when MSCs are cultured sequentially with bFGF, FGF-8, and finally BDNF, a more mature neuronal phenotype is observed since approximately 30% of the cells express markers of dopaminergic neurons, 20% of serotonergic neurons, and 50% of GABAergic neurons [74].
Jin et al. treated murine bone marrow MSCs with neurobasal medium supplemented with B27, glutaMAX, FGF-2, and EGF for seven days and subsequently added RA and β-NGF to the medium for four weeks to induce neural differentiation. This protocol promotes that the treated cells acquire a neuron-like polar morphology. Most of these cells express the mature neuronal markers NeuN and MAP2, and some the microtubule-associated protein tau. Furthermore, cells in these cultures express α 1A and α 1B calcium channel subunits associated with neuronal calcium channels and the protein synaptophysin. Finally, many differentiated MSCs stain positively for GABA and the NR2A subunit of the glutamate NMDA receptor [75].
Tropel et al. used a one-step protocol to differentiate murine MSCs in neurons. Cells were seeded on plates coated with poly-D-lysine and cultured for seven days in DMEM medium supplemented with bFGF. Tow days after the addition of the growth factor, some short neurite-like extensions are observed in MSCs, which fully develop after one week, where the cells acquire a neuron-like morphology. However, the cells revert to a fibroblast shape after a few days. Immunostaining shows that approximately 50% of undifferentiated cells express Nestin, 12.4% NF-L, and 2% β-tubulin III, while treatment for one week with bFGF dramatically increases the number of positive cells to 94.5%., 88.5% and 65%, respectively. In addition, this expression of neural markers is combined with a functional acquisition of neurons, such as an increase in the concentration of cytosolic calcium in response to specific neuronal agonists such as glutamate and veratridine [76].
In 2007 Trzaska et al. used an induction cocktail consisting of SHH, FGF8, and bFGF, to promote neuronal differentiation of bone marrow-derived human MSCs. 12 days after exposure to these factors, MSCs develop a characteristic neuronal morphology and an increase in the expression of neuronal markers NeuN, B-tubulin III, TH, DAT, En1, En2, Wnt1, Lmx1a, Nurr1, and Pitx3, but not glial markers compared to untreated MSCs. Likewise, as cells acquire a postmitotic neuronal fate, the expression of the cell cycle activating proteins cyclin B, cyclin-dependent kinase 2, and the nuclear antigen of the proliferating cell is negatively regulated. The neuronal characteristics of the induced MSCs were confirmed by the expression of the ion channel genes of K+ (Kv 4.2) and voltage-gated Na+ (NaV). Quantitative analyzes indicate that more than 90% of the treated MSCs express NeuN and β-tubulin III, while 68.7% of these cells were TH positive. Likewise, these cells are capable of synthesizing and secreting dopamine into the environment independently of depolarization. Electrophysiological analysis shows that these cells have a resting membrane potential ranging from −50 mV to −70 mV. However, none of the cells exhibit spontaneous and evoked action potentials. Additionally, although MSC-derived neuron-like cells express functional K+ channels, they express deficient levels of voltage-gated Na+ and Ca2+ channels, suggesting that SHH, FGF8, and bFGF-induced MSCs produce Dopaminergic cells that have not acquired the properties of completely differentiated neurons [77].
In 2011 Datta et al. used this induction protocol to differentiate human MSCs from the umbilical cord and bone marrow. Finding that after exposure to SHH, FGF8, and bFGF, MSCs from both sources change morphologically towards neuron-like cells, which upregulate the expression of β-tubulin III, Nurr1, and EN1, while reducing the expression of Nestine, without presenting significant differences between them. Immunohistochemistry reveals that these differentiated cells are positive for the neuronal markers β-tubulin III, Map2ab, and Kv4.2 and the specific markers of dopaminergic neurons TH DARPP32, Nurr1, PitX3, and VMAT2. At the same time, the MSCs Untreated do not exhibit any appreciable staining for these markers. Cellular quantification shows that 91.4% of induced bone marrow MSCs and 91.84% of umbilical cord MSCs are positive for the mature neuronal marker Map2ab, 63.98%, and 65.82% for TH and 62.38% and 68.90% for Nurr1, respectively. For its part, the functional analysis shows that cells induced from both sources release dopamine constitutively, unlike untreated MSCs. ATP stimulation promotes an increase in the release of this neurotransmitter in induced MSCs regardless of its origin [78].
For its part, treatment of human MSCs derived from dental pulp with these factors for three days and subsequent maintenance in a neurobasal medium supplemented with B27 and BDNF without morphogens for six days generates a neuronal-type morphological change in MSCs, thus as a positive regulation in the expression of the specific transcription factors of dopaminergic neurons Nurr1, Engrailed 1 and Pitx3, as well as a decrease in the expression of Nestin, musashi12, and HNK1. Immunofluorescence and flow cytometry analysis shows a higher number of cells positive for dopaminergic neuronal markers in induced MSCs with almost 77% of MSCs positive for TH, 81.92% for En1, 81.21% for Nurr1, and 77.87% for Pitx3. For their part, functional studies indicate that induced MSCs constitutively secrete dopamine and after stimulation with KCl and ATP. At the same time, untreated MSCs do not show any appreciable release of this neurotransmitter. Furthermore, induced MSCs showed an influx of intracellular Ca2+ in the presence of KCl, which is not observed in untreated MSCs [79].
Wang et al. used a cocktail of SHH, FGF-8, GDNF, and forskolin to induce neuronal differentiation of human MSCs derived from dental pulp pre-induced with bFGF and EGF. After adding bFGF and EGF, the cells are grouped into spheres containing positive Nestin progenitors and neuronal cells positive for β-tubulin III but negative for TH. Once the inducers are added to the cultures, some neuron-like cells are generated with multiple neurites expressing β-tubulin III and MAP2. Approximately 10% of the cells stain positively for TH. Real-time RT-PCR analysis indicates that when MSCs are cultured in the induction medium, the transcription levels of Nestin and β-tubulin III are down-regulated compared to uninduced MSCs, whereas a significant increase in transcription levels of MAP2 and TH. Furthermore, as tested by liquid chromatography, it is possible to detect the presence of dopamine in the supernatant of the MSC culture after induction. At the same time, this neurotransmitter is not seen in the supernatant of the non-induced MSCs or spheres [80].
Wang et al. incubated murine bone marrow MSCs with tricyclodecan-9-y-l-xanthogenate (D609), a specific inhibitor of phosphatidylcholine-specific phospholipase C, with or without FGF-2 for two days and maintained the neuronal status of these cells when cultured with FGF-2 for six additional days. The tests show that cell viability increases, and the apoptotic index are significantly lower in cells treated with FGF-2 than those without FGF-2 at 48 h. During the next six days, the differentiated cells show typical neuronal morphology in the presence of FGF-2. However, the number of differentiated cells decreases, and many die without this growth factor. The immunohistochemical assay shows that these cells induced with D609 and FGF-2 show strong positive signals for NSE, NF-L, and synapsin and high mRNA expression levels of NSE and NF-L, compared to undifferentiated MSCs. Likewise, after induction for 48 h, the differentiated cells have a lower membrane potential compared to the undifferentiated MSCs, which becomes more negative when the cells are subsequently cultured with FGF-2 for six days. Neuronal differentiation is accompanied by a significant increase in the change in mitochondrial membrane potential, indicative of mitochondrial hyperpolarization, and a decrease in ROS levels after 6 hours in the group treated with D609 and FGF-2 [81].
Marei et al. Treated adipose tissue human MSCs with FGF2 and heparin for 15 days to induce their neuronal differentiation. This protocol promotes changes in the cell morphology of spindle-shaped MSCs towards cells with spherical morphology and cellular processes. On the other hand, the transcriptional analysis shows that the expression levels of β-tubulin III, MAP2, ChAT, and TH are positively regulated in the induced MSCs compared to the control cells [82].
Mareschi et al. used human MSCs from amniotic fluid to evaluate their neuronal differentiation by culturing them with FGF, EGF, and neural survival factor (NSF-1), for three weeks. Under these conditions, cells aggregate into spheres suspended in the medium, and then adherent clumps form, with cells showing prominent processes that slowly increase during treatment with growth factors. Neurospheres-like floating pools analyzed after three days of culture are positive for Nestin, while cytokeratin expression remains negative and vimentin expression decreases compared to untreated cells. Likewise, neuronal induction increases the expression of neuronal markers in MSCs. In particular, positivity for NSE and MAP2 is observed in 80% of cells. Electrophysiological analyses show that undifferentiated MSCs present transient Na+ entry currents sensitive to tetrodotoxin (TTX) and that treatment with growth factors promote an increase of approximately three times the density of these currents. Likewise, an increase in the expression of the Na+ channel subunits is found in differentiated cells, compared to untreated MSCs [83].
Jang et al. cultured human MSCs isolated from adipose tissue in DMEM medium supplemented with bFGF for seven days, accompanied by the addition of forskolin for an additional seven days to induce their neuronal differentiation. After treatment, most MSCs exhibit distinct bipolar or multipolar morphologies with branching processes, visualized by the immunocytochemistry of the somatodendritic marker, MAP2, and the axonal marker, GAP43. In addition, in neurally differentiated MSCs, immunoreactivity is observed for markers of neural stem cells (Nestin), neuronal (Tuj1, MAP2, NFL, NFM, NFH, NSE, and NeuN), synaptic (GAP43 and SNAP25), astrocytic (GFAP) and Oligodendrocytes (CNPases) very high when cultured in the presence of bFGF and forskolin, while the portion of undifferentiated MSCs expressing specific markers is very low. The RT-PCR analysis shows mRNA levels encoding ABCG2, Nestin, Tuj1, MAP2, NFL, NFM, NSE, GAP43, SNAP25, GFAP, and CNPase upregulated in differentiated MSCs. The functional analysis of these cells shows that more than 75% of the differentiated MSCs acquire functions similar to neurons characterized by the visualization of voltage-dependent sodium currents sensitive to TTX, outward K+ currents, and negative resting membrane potentials. A more detailed examination by RT-PCR shows that undifferentiated MSCs expression channel genes for sodium (SCN5A), potassium (MaxiK, Kv4.2, and EAG2), and calcium channels (CACNA1C and CACNA1G), which are significantly increased by inducing differentiation in these cells. In contrast, the Kv4.3 and Eag1 potassium channel genes and the TTX-sensitive sodium channel gene are only expressed in cells subjected to the differentiation protocol [84].
Ying et al. promoted the neuronal differentiation of murine MSCs from adipose tissue by preincubating these cells in a DMEM medium, supplemented with EGF, bFGF, and BDNF for three days. The subsequent addition of indomethacin, insulin, and IBMX for 48 h for their induction. After treatment, MSCs show changes in cell morphology, including cytoplasmic contraction, axon formation, dendrite-like cytoplasmic projections, and express neuronal and glial markers Tuj-1 and GFAP, respectively, which are extremely low before induction. Quantitative analysis indicates that approximately 70% of the induced cells are positive for Tuj1, and 75% are positive for GFAP. However, the absence of BDNF in pre-induction reduces the number of positive cells for these markers to 45% and 50%, respectively [9].
In 2014, Feng and collaborators planted adipose tissue-derived human MSCs in gelatin-coated plates. For neuronal differentiation, cells were pre-induced with β-Me and bFGF for eight days. Subsequently, culture in a DMEM/F12 medium supplemented with L-glutamine, B27, N2, and β-Me for seven days, to finally treat them with EGF and bFGF for an additional seven days. MSCs subjected to this protocol exhibit distinct morphologies similar to neuronal stem cells, which can easily detach from the background after digestion and merge to form neurospheres when cultured in ultra-shallow dishes. Furthermore, these cells cultured as monolayer or neurospheres highly express Sox1, Pax6, Nestin, and Vimentin, compared to undifferentiated cells. Subsequently, they tested the terminal differentiation capacity of the cells subjected to this protocol by seeding the cells on coverslips covered with poly-D-lysine and laminin in a Neurobasal medium supplemented with N2, FBS, horse serum, and RA for two weeks, with the subsequent administration of BDNF for neuronal induction. After two weeks of induction, cells cultured in a neuron induction medium show a similar structure to neurons and MAP2 expression. These cells exhibit a TTX-sensitive voltage-dependent sodium current, exhibiting a rapid on-off characteristic. In addition, the expression of the sodium ion channel genes (SN5A, NE-NA), the potassium ion channel genes (MaxiK, KV4.3, KV4.2, KV1.4, EAG1, EAG1), and calcium ion channel genes (CACNA1C, CACNA1G) are increased in terminally differentiated MSCs compared to undifferentiated MSCs [85].
In that same year, Yu et al. compared the neuronal differentiation capacity of human MSCs obtained from adipose tissue, bone marrow, and umbilical cord when cultured in the presence of BDNF. After seven days of induction, human MSCs assume a neuron-like morphology regardless of the acquisition site, while control MSCs retain fibroblast morphology. Likewise, all the MSC groups treated with BDNF show higher levels of Nestin expression than the control groups, without significant differences between them. On the other hand, a higher expression of ChAT and GAD67 is observed in the induced bone marrow and umbilical cord MSCs compared to control MSCs, and adipose tissue induced MSCs. Exposure to BDNF appears to promote GABAergic neuronal differentiation since an increase in the percentages of GABA+/DAPI+ cells is observed in treated MSCs, compared to control cultures in adipose tissue MSCs (9.7% vs. 5.3%), bone marrow (21.5% vs 8.4%) and umbilical cord (7.0% vs 1.8%), respectively [86].
Recently Jahan et al. studied the neuronal differentiation of umbilical cord-derived human MSCs by treating them with NGF. 4 days after the addition of this growth factor, morphological changes similar to the neuronal type are observed, such as rounding of cells and the development of neurite-like extensions. These neuron-like cells show a significant and gradual increase at the messenger and protein level of the neuronal markers NF-M, β-tubulin III, and PSD-95 compared to undifferentiated MSCs [87] (Supplementary TableS4).
10. Coculture
Sánchez-Ramos et al. carried out the coculture of MSCs previously differentiated by RA and BDNF with fetal primary neuronal cultures to increase the neuronal differentiation of these cells. After co-cultivation, 2% - 5% β-galactosidase-labeled MSCs stain positively for NeuN, and 5% to 8% do so for GFAP, a much higher proportion than observed when the MSCs are cultured alone. Most GFAP+ cells labeled with β-galactosidase have an oval or spindle-shaped morphology with short processes similar to those of NeuN+ cells. However, some GFAP+ cells show an irregular shape that approximates the morphology of glial cells [88].
Joannides et al. induced neuronal differentiation of bone marrow-derived human MSCs by exposure to EGF and FGF-2 for seven days, followed by treatment with postnatal astrocyte conditioned medium derived from rat hippocampal cultures. Twenty-four hours after the addition of mitogens, morphological changes characterized by cell retraction are observed. At the same time, at the end of 7 days of induction, the co-expression of the neural markers β-tubulin III and pan-neurofilament is evident in the soma of a subpopulation of rounded cells. Upon removal of mitogens, the expression of these markers is significantly reduced with an associated increase in cells expressing smooth muscle actin co-localized with FN. However, treating differentiated cells with an astrocyte-conditioned medium promotes an increase in the number of cells expressing neurofilament and β-tubulin III compared to treatment without the conditioned medium and down-regulation fibronectin in these cells. Furthermore, these positive β-tubulin III cells show greater elaboration of processes than cells that are only treated with mitogens [89].
Fu et al. use umbilical cord-derived human MSCs in a neuron-conditioned medium, observing that MSCs acquire a neuronal-like morphology on day 12. The processes generated in the MSCs are excessively long and form cell-cell contacts, creating a network’s appearance. Likewise, these neuron-like cells show an increase in the expression of NeuN and NF compared to the untreated MSCs. The quantitative study reveals that on the ninth day of coculture, 58.2% and 87.4% of MSCs stain positively for the neuronal markers NeuN and NF, respectively. In comparison, only 1.95% and 1.77% do so for GFAP and OX42, respectively. Furthermore, treatment with the conditioned medium induces in some MSCs the expression of functional calcium-binding proteins, such as parvalbumin and calbindin. Finally, the kainate receptor subunits, including GluR6, GluR7, and KA2, begin to appear in small amounts in MSCs after coculture for six days, and the labeling becomes significant after 12 days, allowing these cells to respond to a glutamate bath, generating input currents [90].
This working group induced human MSCs isolated from Wharton’s gelatin to transform into dopaminergic neurons in vitro by treatment with the neuron-conditioned medium for nine days, with subsequent exposure to SHH and FGF8 for an additional 12 days. Immunocytochemical staining shows that MSCs treated with this protocol are TH positive with a success rate of 12.7%, capable of synthesizing and releasing dopamine to the culture medium. On the other hand, staining for the enzyme glutamic acid decarboxylase indicates that MSCs exposed to conditioned medium and morphogens differentiate, in addition to dopaminergic neurons, in low yields of GABAergic cells [91].
Zurita et al. compared the differentiation efficiency of murine bone marrow MSCs employing a chemical protocol based on the use of β-Me as pre-inducer and DMSO, BHA, KCl, valproic acid, and forskolin as inducing agents, with a method based on co-culture of the MSC with Schwann cells. One hour after starting the chemical induction, approximately 30% of MSCs show positivity for Nestin, this percentage increasing at four h (64%) while showing morphological changes related to neuronal cells. One week after starting the chemical induction, positivity for NSE is found in 79.9% of MSCs, while 85.3% show positivity for NF-200 and 86.2% for β-tubulin III. For its part, it is observed that co-culture induces late neuronal differentiation since, although MSCs begin to express Nestin 4 h after coculture, most MSCs need approximately one week to show typical neuronal morphology. However, by this time, the neuron-like cells no longer expressed Nestin but showed positivity for NSE (60%), NF-200 (52%), and β-tubulin III (34%) [92].
Since cell fusion cannot be excluded as the cause of apparent transdifferentiation of MSCs cultured in Schwann cells, these authors carried out co-cultures of bone marrow MSCs and Schwann cells in the presence of polycarbonate membranes to prevent direct contact between these cells. Under these conditions, MSCs express Nestin 4 h after starting the cocultures. A strong expression of neuronal markers such as NF-200, β-tubulin III, or GFAP is revealed at 72 h, increasing during the second week of treatment. At the end of the second week, most MSCs show clear neuronal morphology, and around 9% of MSCs show positivity for Nestin, 39% for NF-200, and 43.2% for β-tubulin III, while only 20.4% of cells are positive for GFAP [93].
For their part, Krampera et al., Cocultivated MSCs of different origins with Schwann cells to induce their neuronal differentiation. After 12 days of coculture, a proportion of MSCs acquires the morphological characteristics of Schwann cells and a selective expression of the markers S-100 and PMP-22. The ratio of these cells depends on the origin of the MSCs. MSCs derived from adipose tissue show the highest percentage of cells that acquire the Schwann cell phenotype after seven days of coculture. This proportion is significantly lower than the use MSCs of the thymus, spleen, and particularly bone marrow. On the other hand, in this study, it is observed that direct contact between the Schwann cells and the MSCs is necessary to induce the morphological and phenotypic changes of the MSCs, since the treatment of the MSCs with the cell culture supernatant Schwann’s does not generate the effects above. Finally, Kamprera et al. show that irradiation of Schwann cells before coculture does not change the proportion of differentiated MSCs, thus ruling out an essential role of cell fusion in expressing glial markers by MSCs [94].
Wislet et al. generated Nestin-positive murine bone marrow MSCs by EGF treatment. Subsequently, Nestin positive MSCs were co-cultured in a conditioned medium with neurons from mouse cerebellar granules to induce their terminal differentiation. After five days of coculture, 40.17% of the MSCs stain positively for GFAP, 19% for Tuj1, and 19.30% for NeuN. However, these neuronal markers cannot be detected when coculturing Nestin negative MSCs or Nestin positive MSCs, cultured physically spaced neurons. Electrophysiological assays show that neuron-like cells have a resting membrane potential of −55.7 mV at ten days of coculture and that these cells express functional voltage-gated K+ channels in the early stages of differentiation, followed by the presence of voltage-gated Na+ channels, which allows them to trigger action potentials, unlike control MSCs. Finally, it was shown that these differentiated cells could respond to various neurotransmitters such as GABA, glycine, and glutamate by evoking electrical currents that are reversibly blocked by specific antagonists of their respective receptors [95].
Nivet et al. demonstrated that coculturing human MSCs from the olfactory epithelium with mouse hippocampal slices provides a suitable environment for the in vitro neuronal differentiation of these cells, since three weeks after engraftment, surviving MSCs are observed on top of organotypic cultures of the hippocampus, where some of them show a morphology similar to an interneuron with the expression of MAP2, but not GFAP [30].
Recently, Lo Furno et al. induced neuronal differentiation of human MSCs from adipose tissue by treating them for seven days with a conditioned medium obtained from cultures of olfactory envelope cells or Schwann cells. MSCs grown under these conditions have a more complex morphology than MSCs grown alone. Likewise, these cells have a higher immunoreactivity for Nestin, MAP2, and Synapsin than control MSCs. In particular, a more pronounced increase in MAP 2 positivity is detected when using the olfactory envelope cell-conditioned medium relative to the Schwann cell-conditioned medium. To glial differentiation, the immunopositivity of GFAP is relatively low in controls but is markedly increased in cells treated with the conditioned medium, especially when using a medium derived from Schwann cells [96].
Finally, in 2019, Wei et al. cultured bone marrow-derived murine MSCs with Schwann cell-conditioned medium to induce neuronal differentiation. Upon treatment with the medium, the shape of the MSCs changes to a neuronal-like morphology as cell bodies gradually shrink and neurites form. Furthermore, an increase in Nestin and MAP2 markers are detected in neuron-like cells. The microRNA analysis identifies 83 microRNAs significantly differentially expressed between the co-cultured group and the control group, where the Gene Ontology analysis indicates the enrichment of microRNA target genes for the development of neuronal projection, the regulation of axon genesis, and the positive regulation of proliferation in MSCs cultured with the conditioned medium. Furthermore, the analysis of the KEGG pathway shows that the Hippo, Wnt, and SHH signaling pathways are potentially associated with the neuronal differentiation of MSCs treated with this medium [97] (Supplementary TableS5).
11. Gene Transfection
A small interfering RNA (siRNA) directed against notch-1 (mNotch-1shRNA) was transfected into CMM. Its neuronal differentiation was induced when cultured in a Neurobasal medium supplemented with RA and BDNF for six days. After 24 h of transfection, the MTT assay values of mRNA Notch-1 in MSCs decrease and are much lower than those of non-transfected MSCs. Likewise, there are more apoptotic cells in the transfected MSCs than in the control CMM. On the other hand, the transfection of mNotch-1shRNA alone is not enough to induce the differentiation of MSCs in neural cells. However, after induction with RA and BDNF, the transfected MSCs undergo a remarkable morphological change, where 24 h after induction, the cytoplasm of these cells retracts and presents extensions in the form of processes, which improves during the following six days. Likewise, the expression of NSE and NF200 increases to high levels in the mNotch-1shRNA MSCs after induction. In contrast, the expression of GFAP decreases in this group compared to the induced MSCs without transfection [98].
In 2008, Trzaska et al. Sought to improve the neuronal differentiation obtained in 2007, transfecting an RNAip directed to REST, a transcriptional regulator that silences the expression of specific genes of mature neurons in non-neuronal cells and neuronal progenitors, before undergoing the differentiation with SHH, FGF-8, and bFGF. The bright-field images taken on days 6 and 12 of induction show a more mature phenotype in REST knockdown cells compared to control transfected cells. Furthermore, the loss of the REST protein allows a higher expression of TH, NeuN, Pitx3, and Nurr1 and an increase in NaV protein levels in neuronal cells derived from MSCs. On the other hand, the electrophysiological tests show that the induction at day 12 in MSCs with a drop in REST generates the presence of spontaneous solid discharges and postsynaptic currents compared with the control cells [99].
Yang et al. used lentiviral vectors to deliver siRNA against REST1 (siREST) or negative control (siControl) to differentiate bone marrow human MSCs into a neuronal phenotype. Fourteen days after transfection, most siREST MSCs exhibit a neuron-like morphological change, with structures equivalent to long neurites and Nissl bodies, but not in siControl MSCs. Cell proliferation analysis shows that MSC-siREST grows slower than MSC-siControl, suggesting that MSC-siREST is in a differentiation stage. The RT-PCR analysis shows that at 14 days of transfection, the MSC-siREST increases the expression levels of neuronal genes BDNF, NGN-1, NSE, SYP, and SCG10 while the expression of these genes does not it is detectable in the CMM-siControl. Likewise, these cells stain positively for β-tubulin III, NSE, MAP-2, and NF-200, without labeling glial markers GFAP and Olig2. Finally, these neuron-like cells show TTX-sensitive NA (+) currents and action potentials, which are not observed in MSC-siControl, which only present outward K+ currents [100].
Barzilay et al. Used the delivery of lentiviral genes to exogenously administer the homeobox one beta LIM (LMX1b) transcription factor to bone marrow MSCs before differentiation with FGF-2 FGF-8, and SHH. Before induction, no change is observed in the expression levels of transcripts of typical midbrain dopaminergic genes such as MSX1, Sox2, Neurogenin 2, hASH1, En1, or Pitx3 in LMX1a transfected MSCs, similar to MSX transfected with a GFP control. However, after three weeks of induction, an impressive number of these factors are up-regulated in LMX1a MSCs compared to control MSCs. Furthermore, after differentiation, more than 90% of LMX1a MSCs express Tuj1 compared to the baseline level of Tuj1 expression in undifferentiated MSCs. Some differentiated LMX1a MSCs stain positively for MAP2 and synapsin, and some express voltage-gated sodium channels typical of neurons. On the other hand, the positive regulation of the TH protein promoted by induction is significantly higher in LMX1a MSCs than control transfected MSCs after induction, which allows LMX1 MSCs to secrete three times more dopamine into the medium than induced control MSCs [101].
For their part, in 2013, Yan et al. Induced the ectopic expression of LMX1a and Neurturin in MSCs before their neural differentiation through exposure to β-Me, RA, bFGF, FGF-8, and SHH. After 21 days of induction, the transcriptional levels of TH, Msx-1, Nestin, MAP-2, Lmx1β, Foxα2, Pitx3, DAT, β-tubulin III, Nurr1, and EN1 are significantly upregulated in transfected and induced MSCs, compared to control induced and transfected MSCs, and this was confirmed at the protein level by immunofluorescence analysis, where the results reveal staining for NSE, MAP-2, β-tubulin III, and TH in transfected MSCs as opposed to control MSCs [102].
In the same year, Wang et al. transfected murine bone marrow MSCs with a siRNA against Caveolin 1 before their neuronal differentiation with β-Me. Transfection alone did not generate significant morphological changes in MSCs. However, the levels of Notch-1, the intracellular domain of Notch (NICD), and Hes5 were markedly down-regulated in these cells after the transfection process. After treatment with β-Me, most cells present a typical neuronal appearance at six days of induction. Unlike other tests, in this study, the addition of β-Me generates an expression of Map2 at the mRNA level and protein and NSE. These effects on the morphology and the expression of neuronal proteins are increased in the transfected group. In addition, after a 6 h induction, the expression of caveolin-1 is positively regulated in both the transfected and control MSCs. At the same time, the levels of Notch-1, NICD, and Hes5 decreased significantly, particularly in the group of transfection with siRNA CAv1 [103].
Park and colleagues used a 2-step protocol to differentiate human MSCs into motor neurons. First, the ectopic expression of the Olig2 and/Hb9 genes was promoted in MSCs by delivery by viral vectors, to later treat these cells with β-Me, forskolin, RA, bFGF, and SHH for their differentiation. Treatment with these factors significantly increases NF-M mRNA levels in both transfected and non-transfected cells compared to untreated cells. However, the mRNA for Islet-1 and VAChT, markers of motor neurons, only increase when transfected cells are induced. After nine days of exposure to the induction medium, approximately 32% of the transfected cells adopt a neuron-like morphology, of which 18% maintain neuronal morphology even after two days of the removal of the induction medium. Electrophysiological assays demonstrate that the resting membrane potential of cells transfected and treated with induction medium is more negative than that of transfected non-induced MSCs. More than half of these cells exhibit evoked action potentials. Furthermore, these transfected and induced MSCs can form functional connections with muscle fibers in vitro, as a motor neuron would [104].
Differentiation of human MSCs was induced by gene transfection of NICD followed by culture in α-MEM medium supplemented with forskolin, bFGF, and ciliary neurotrophic factor (CNTF). After transfection, MSCs show increased glutamate transporter GLAST and Nestin expression compared to untransfected MSCs. Five days after induction, the transfected and induced MSCs show neuron-like processes and express the neuronal markers MAP-2ab, NF-M, and β-tubulin III. Immunohistochemical assays show that more than 90% of the transfected MSCs stain positively for MAP-2ab, of which 89.4% also stain positively for NF-M and β-tubulin III after differentiation, while GFAP cells are not detected. Electrophysiological analysis shows that transfected and induced MSCs have a more negative resting membrane potential than control transfected MSCs. An increase in rectifying potassium current is seen in 58% of transfected and trophic factor-treated MSCs, but not fast voltage-gated sodium currents. Finally, the addition of GDNF to these cells increases the expression of Nurr-1, Lmx1b, En1, Ptx3 and increases the proportion of cells immunopositive for TH (41.0%), compared to transfected and induced MSCs without GDNF (3.9%), which allows them to synthesize and secrete dopamine to the culture medium in response to depolarizing stimuli [105].
In 2015, Liu et al. Transfected the human BDNF gene into guinea pig-derived MSCs and treated them with RA for neuronal differentiation. After culturing for 48 h with RA, the viability of transfected MSCs is greater than that of induced or non-induced non-transfected MSCs. Said cells transfected and exposed to RA have a morphology similar to a neuron, compared to untreated MSCs. Immunohistochemical and RT-PCR assays show that transfected MSCs express higher levels of nestin, BDNF, NSE, and GFAP, compared to non-transfected MSCs regardless of whether they were treated with RA or not. However, more cells positively co-stained for nestin, NSE and GFAP are detected in transfected MSCs subjected to differentiation than in non-induced ones [106] (Supplementary TableS6).
12. Micro-RNA
Jing et al. Transfected murine bone marrow MSCs with a viral vector containing the sequence of microRNA-9, and subsequently, the MSCs were induced into neurons by exposure to β-Me. In principle, the infected MSCs show a lower survival than the group transfected with empty vector, however after induction, the transfected MSCs show higher levels of MAP2 at both the RNA and protein levels, as well as a lower expression of Notch1, which induced cells without transfection [107].
For their part, Wu et al. Analyzed the action of mir-125-b in the neuronal transdifferentiation of murine bone marrow MSCs. They transfected them with a mimic miR-125b (Mimic), anti-miR-125b (group inhibitor), or without transfecting before its differentiation with β-Me. As expected, the relative folding of miR-125b increases significantly in the Mimic group but decreases considerably in the Inhibitor group. Neuronal differentiation, induction for six days, increases the levels of β-tubulin III, MAP-2, NF, NSE, GFAP, and Nestin at the messenger and protein level in the three experimental groups compared to the messenger and protein to the untreated group. However, the relative quantification of mRNA of these markers is significantly increased in the Mimic group compared to the untransfected β-Me group but significantly decreased in the inhibitor group. In addition, the induced MSCs of the mimic group acquire a morphology more similar to nerve cells for the untransfected cells. In contrast, in the inhibitor group, the morphological changes are not so noticeable and occur in only a few cells [108].
Duan et al. Infected MSCs with a lentivirus containing a miR-29a inhibitor before its induction with β-Me, DMSO, and BHA, finding that the drop in mir-29a promotes an increase in REST expression levels. There was a decrease in SNAP25, L1CAM, and the neuronal markers NES and Tau compared to the CMM induced without transfection. On the other hand, by treating these cells with a siRNA against REST, the expression of NSE and Tau is rescued, suggesting that miR-29a regulates neurogenic markers by targeting REST in induced MSCs [109].
On the other hand, Han et al. found that after exposing MSCs to these inducing factors, the expression of miRNA let-7f-5p is negatively regulated, while the expression of Par6α, a component of the Par3/Par6/aPKC complex, increases. Likewise, computerized analysis and luciferase assay show that Par6α is a target gene for let-7f-5p. Using gain and loss of function approaches demonstrated that Par6α and MAP2 expression are inversely correlated with the levels of let-7f-5p during differentiation. On the other hand, the silencing of Par6α using siRNA attenuates the neuronal differentiation of MSCs by decreasing the levels of MAP-2, suggesting that inhibition of let-7f-5p facilitates MSC induction in neuron-like cells by targeting Par6α directly [110].
Takeda & Xu treated MSCs with exosomes derived from PC12 cells, a neuronal cell line, for one week to test their inducing power. Said exosomes were isolated from PC12 cells in different stages of differentiation with NGF, calling the exosome of undifferentiated PC12 cells “exosome D0”, and the exosomes of PC12 cells treated with NGF for 3 and 9 days “D3” and “D9”, respectively. On the other hand, as a control, MSCs were treated with exosomes derived from the B16-F10 melanoma cell line. After treatment with exosomes derived from the PC12 cell line, MSCs present neurite-like extensions, which are not observed in untreated MSCs or treated with B16-F10 exosomes. Likewise, the expression of MAP2 and NSE is positively regulated after treatment with exosomes D3 and D9.
Exosomes derived from the PC12 cell line were used to evaluate the possible differentiation mechanisms promoted by the miRNAs contained in the exosomes. There were 101 miRNAs in the exosome samples using microarrays, of which nine are positively regulated after treatment with NGF, compared to the exosomes of B16-F10 cells. In particular, the expression of miR-125b is 319 times higher in the D9 exosome compared to the B16-F10 exosome, which suggests that this miRNA, together with other components of the exosomes of neural cells, may play a role in the expression of neuronal markers in MSCs. In contrast, exosomes derived from B16-F10 cells and exosome D0 do not produce changes in the expression levels of these markers [111].
13. Small Molecules
In 2017 Alexanian et al. used their 2010 differentiation protocol together with the small molecules SB431542 and dorsomorphin (DM), two specific SMAD inhibitors that inhibit activin/nodal/TGF-β signaling pathways bone morphogenic protein (BMP), respectively, to induce neuronal differentiation of human MSCs. After three weeks of induction, MSCs experience increases in various neuronal genes such as Sox2, Map2, β-tubulin III, NSE, Nurr-1, ChAT, TH, NF, VGLUT1, SYP, SYN-1, VGAT, GAD67, and PITX3, relative to untreated MSCs. Approximately 95% of the total cells subjected to differentiation present neuronal morphology, while the rest, although they present fibroblastic morphology, still express several neural markers. The cell count positive for specific neuronal markers shows that approximately 68.05% of the cells stain for TH, 89.12% for Nurr1, and 11.03% for ChAT. Synaptophysin immunostaining reveals synapse-like structures that form between differentiated cells [112].
Dual inhibition of small molecule-mediated activin/nodal/TGF-β and BMP signaling pathways induces neurite outgrowth in MSCs after 14 days of exposure. It promotes increased expression of Pax6 transcription factors and Sox1 and the expression of NF200, NES, and β-tubulin III. This process correlates with the activation of p38, Erk1/2, PI3K, and Akt signaling since enhanced phosphorylation of Erk, PI3K, and Akt is found in cells subjected to dual inhibition. Three years later, Madhu et al. used these two small molecules without adding any other factor as inducers in the neuronal differentiation of human MSCs derived from adipose tissue. The presence of NSE further confirmed the induction of a neuronal phenotype on day 14 in the cells treated with the inhibitors, where more than 85% of the MSCs treated with SB and DM stain positively for this neuronal marker [113].
J. Park et al. developed an optimal protocol for inducing adipose tissue-derived human MSCs in neuronal stem cell-like cells with more than 85% efficiency using small molecules. To do this, they treated bFGF pre-induced MSCs with three inhibitors of the BMP signaling pathway: SB431542, noggin, and LDN193289 for eight days. These cells were subsequently cultured for five days in a Neurobasal medium with the presence of ascorbic acid, B27, and N2, to finally replace the medium with a medium supplemented with EGF and bFGF for an additional seven days. The qRT-PCR analysis shows that the expression levels of the markers nestin, Sox1, Sox2, Pax6, Musashi1, vimentin, EMX1, Gli3, FoxG1, Tuj1, and Olig 2 are significantly increased in MSCs cultured in the presence of small molecules, compared to CMM without the addition of these. These cells grow as neurospheres in suspension culture. Flow cytometry analysis shows that the proportion of cells positive for NCAM, Nestin, and Ki67 is 85.4%, 92.9%, and 76.8%, respectively. Subsequently, these authors seeded the partially differentiated cells in a neuronal induction medium composed of Purmorphamin (SHH activator) and BDNF to support the maturation of these cells. After seven days of culture, the cells exhibit neuron-like structures characterized by distinctive bipolar or multipolar neurite outgrowth at the periphery of their cell bodies. The qRT-PCR analysis reveals that the cells show increases in the expression levels of TuJ1, MAP2, Dlx5, Lhx6, GAD67, and the sodium ion channel SCN5A, seven days after the addition of these factors. Quantitative analysis reveals that more than 75% of cells stain doubly positive for the immature neuronal markers TuJ1 and Pax6 and the mature neuronal markers MAP2 and NeuN.
In contrast, only 33% of cells express the astrocyte markers GFAP and S100 and 17% of oligodendrocytes OLIG2. Electrophysiological testing of these cells shows that neuron-like cells successfully elicit action potentials. Some cells show a depolarizing dip in membrane voltage produced to hyperpolarizing current injection. Furthermore, a spontaneous postsynaptic current is detected in the recorded cells, indicating that these cells are connected by synapses and form a neural network. Finally, it was shown that treating MSCs exposed to small molecules with dbAMPc and BDNF for 14 days promotes a significant increase in the expression levels of genes related to GABAergic neurons such as GABRA2, GABRA5, vGat, and GAD65, while immunostaining reveals that 67% of MAP2-positive neuron-like cells also express GAD and 47% doubly stain for MAP2 and GABA. The functional study of these cells shows that the cells exhibit electrophysiological characteristics of active GABAergic neurons, such as a fast Na (+) influx current during the depolarization of the membrane potential and an inhibitory postsynaptic current in the presence of NMDAR and AMPAR agonists [114].
In 2019 Hu et al. Used the ROCK kinase inhibitor Fasudil to differentiate murine MSCs from bone marrow pretreated with bFGF. The small molecule induces morphological changes in the MSCs corresponding to neurons. The MSCs shrink from their fusiform shape to a round morphology with long and thin processes, forming networks and contacts with other cells. Compared to the control group, these neuron-like cells increase at the messenger and protein level of the neuronal markers NSE, nestin, and NF-M. Quantitative analysis shows that the positive mean rate of immunofluorescent staining is 69.35% for NF-M, 76.52% for NSE, and 78.95% for nestin. The differentiating effects of fasudil are partially inhibited with an inhibitor of the Wnt/β-catenin pathway (XAV939), suggesting that the differentiation promoted by fasudil is probably due to the activation of this pathway [115].
14. Retinoic Acid
Sánchez-Ramos et al. carried out neuronal differentiation of human umbilical cord MSCs by exposing them to NGF and RA, a small molecule that functions as a ligand for the retinoic acid receptor. Treatment with this molecule promotes increased expression of the neural markers Musashi-1, β-tubulin III, Pleiotrophin, Nestin, Necdin, NeuN, and GFAP, which are expressed at relatively low levels of undifferentiated MSCs. Immunohistochemical analysis shows that 6.2% of the induced cells stain positively for Musashi-1, 18.7% for β-tubulin III, and 66.2% for GFAP. Concomitant with the increased expression of these markers, there is a decrease in the expression of genes associated with the development of blood cell lines. DNA microarray analysis shows that MSCs grown in the presence of RA and NGF exhibit significant changes in the expression of 322 genes out of a total of 12,600 human genes represented on the DNA chip. And at least 20 can be bound to products found in developing neurons, glia, or neural cells [116].
Locatelli et al. induced the formation of spheres from murine bone marrow MSCs by their treatment with bFGF and EGF, similar to the neurospheres of the brain, of which 90% are positive for nestin. These spheres were then immunomagnetically selected to isolate Sca-1 and Thy-1 positive cells, subjected to differentiation with RA and SHH for 7 days. After induction, these cells present a polarized form (unipolar or bipolar) with long cytoplasmic processes. Approximately 40% show immunoreactivity for β-tubulin III and NF while expressing β-tubulin III, NSE, and mRNA at the level of the NF light chain [117].
In addition, murine bone marrow CMMs were treated in DMEM pre-induction medium supplemented with FBS, FGF2, B27, forskolin, and IBMX for 24 h, to then induce their differentiation in DMEM medium supplemented with FGF2, insulin-transferrin-selenium supplements, B27, Forskolin, IBMX, and BDNF. Finally, cells were exposed to a combination of SHH and RA to improve neuronal differentiation. After treatment with SHH and RA, substantial increases in cells with neuronal morphology are observed. MSCs show a variety of neuronal antigens, including MAP2, TUJ1, Tau, NSE, NeuN, and NF, which increases the expression of the glutamate transporters VGLUT1 and VGLUT2, GluR3, and GluR4 receptors. It should be noted that the individual application of SHH or RA promotes little or no effect on the expression of these markers [118].
Cho et al. treated human bone marrow MSCs with a single dose of RA, generating MSCs with neuronal morphology by the seventh day of exposure. At this time point, the treated cells show a high positivity for MAP2 and a slight fluorescence for synaptophysin, which increases by day 12 of treatment. Additionally, this treatment generates that 80% of the cells are positively marked for neurofilament, MAP2, and nestin and that a large proportion of the cells subjected to differentiation generate action potentials [119].
Hermann et al. generated spheres derived from human MSCs from bone marrow donors young (18 - 35 years) and old (45 - 76 years) by treatment with EGF and FGF-2, which differentiate in neurobasal medium supplemented with RA. This study revealed that after inducing differentiation in MSCs from old donors, more than 80% remain positive for fibronectin (FN), while no expression of MAP2, GalC, or GFAP is observed. Furthermore, only 33% of cells stain positively for β-tubulin III and 12% for nestin. In contrast, 42% of the treated cells derived from young donors are β-tubulin III positive, 6% MAP2 positive, 13% GFAP positive, and 15% GalC positive [120]. Likewise, these authors demonstrated that neurospheres treated with RA could undergo terminal neuronal differentiation by adding BDNF to the medium for 14 days. This modification causes a decrease in FN expression levels in the MSCs, NEUROD1, NGN2, and OTX1 while upregulating the expression of the early neuroectodermal gene MSI1. Furthermore, this protocol leads to a mature neuronal phenotype demonstrated by the expression of MAP2ab in about 6% of the cells and an increase in TUBB4/III, SNCA, and TH, as well as an increase in the expression of GFAP and MBP [121].
Gong et al. used RA as a pre-inductive agent for neuronal differentiation of murine bone marrow MSCs for 24 h before culturing them in a differentiation medium consisting of DMEM/F12 medium supplemented with DMSO, butylated hydroxyanisole, KCl, valproic acid, forskolin, hydrocortisone, and insulin for 24 h. After induction, MSCs transform into neural-like cells exhibiting distinct neuronal morphologies, including widely branched single, large, and multipolar, bipolar appearances. The neuronal differentiation efficiency of MSCs pretreated with RA is 83.17%, significantly higher than that of MSCs without pretreatment (61.31%). Likewise, pre-induced MSC-derived neural-type cells exhibit longer axonal length and more neurites. Together the mRNA and protein levels of the Nestin, NSE, MAP-2, Tau, and Tuj1 markers are higher in pre-induced cells. Electrophysiological analysis shows that RA-untreated MSC-derived neural-type cells hyperpolarize after neuronal induction compared to uninduced cells. However, a more significant effect is observed in cells treated with the small molecule. On the other hand, after stimulation with a high concentration of K+, there is a more significant increase in the intracellular calcium concentration in the induced cells than in the undifferentiated cells. At the same time, this effect is enhanced by pretreating the cells with RA [122].
Wang et al. showed that RA could induce efficient neural-type differentiation of bone marrow-derived murine MSCs. With the generation of neuron-like cells, increased expression of neuron-specific markers such as NSE, NF-H, β-tubulin, and MAP-2 and the gradual decrease in the resting membrane potential of these cells after RA treatment. Furthermore, these authors found that myocardin-related transcription factor A (MRTF-A) is activated and translocated to the nucleus during RA-induced neural-type differentiation in MSCs. At the same time, the fall of MRTF-A significantly prevents the up-regulation of neuronal-specific gene expression in response to RA, partially suppressing the differentiation of these cells [123].
Gao et al. used RA to induce neuronal differentiation of MSCs derived from the yolk sac of a chicken embryo, observing that after the induction process, some of the cells show an elongated neuronal morphology with extended processes, as well as an increase in the expression of Nestin and Map-2, which are expressed at deficient levels in undifferentiated MSCs [124].
Recently Lu et al. induced the differentiation of human MSCs derived from the olfactory mucosa into photoreceptor cells by adding EGF, Taurine, and RA for ten days. Treatment with these factors leads to a substantial change in the cellular morphology of cells derived from the olfactory mucosa, where some cells show a spherical shape, an extended bulge, and the presence of primary and secondary branches. Immunofluorescence staining and western blot analysis indicate the presence of the rhodopsin protein, a positive marker for cells expressing induced photoreceptors [125] (Supplementary TableS7).
15. Advantages and Disadvantages of Multiple Differentiation Protocols
The choice of the optimal differentiation method must be made in a particular way considering several factors such as price and the intrinsic characteristics of the cells used to induce the differentiation process. The different methodologies reviewed show considerable variations in differentiation efficiency, maturation, neuronal marker expression, functionality, and cell survival. However, each procedure presents a series of favorable and unfavorable characteristics compared to each other. Table 1 summarizes the advantages and disadvantages of each differentiation method.
![]()
Table 1. Advantages and disadvantages of multiple differentiation protocols used to promote neural differentiation of MSCs.
16. Conclusion
The ability of MSCs to differentiate into neural lineages makes them a promising and a potential tool to generate new advances in the field of cell therapy and regenerative medicine. This review summarized and compared multiple effective methods to induce this differentiation. However, differentiation can vary depending on the site of collection, the methodology used, the physiological state, and the individual’s age from which they were isolated. There is a need for current research and procedures that could provide new insights for a better understanding that could help develop and design new therapies for treating neurological and neurodegenerative diseases.
Supplementary Material: Chemicals Compounds
![]()
Table S1. Breakdown of publications in which antioxidants are used to induce neuronal differentiation of MSCs.
![]()
Table S2. Breakdown of publications using substances that increase cAMP levels to induce neuronal differentiation of MSCs.
![]()
Table S3. Breakdown of publications using chromatin modifiers to induce neuronal differentiation of MSCs.
![]()
Table S5. Breakdown of publications in which coculture with mature neuronal cells is used to induce neuronal differentiation of MSCs.
![]()
Table S6. Breakdown of publications using gene transfection to induce neuronal differentiation of MSCs.
![]()
Table S7. Breakdown of publications in which small molecules are used to induce neuronal differentiation of MSCs.