Suprafibrillar structures of collagen, evidence for local organization and auxetic behaviour in architectures ()
1. INTRODUCTION
The suprafibrillar arrangement of collagen fibrils is often regarded as being at most a lateral packing of local order; however, fibril architecture is a major contributor to the exquisite fidelity of tissue properties and the mechanisms for controlling fibril disposition is poorly understood. There is some indirect evidence from a number of sources that axial interfibrillar registration can occur in collagen [1], and fibrillin rich microfibrils have regions of staggered fibrils within an intact tissue [2]. This type of interaction has implications for the local registration, spatial relationship and mechanical performance of a tissue. In addition, it is critical to know if this is a factor in the modulation of axial data intensity for the modelling of individual collagen fibrillar structures from X-ray diffraction data. Cornea is a unique collagen material reliant on interfibrillar interactions for its high almost perfect transparent properties and extraordinary mechanical resilience [3,4]. Here, the ability of the cornea to transmit at least 99% of incident light is due to the highly regulated sub optical fibrillar size and suprafibrillar organisation of the lamellae [5,6]. The factors that direct and maintain the interfibrillar relationship have been mostly reported on the lateral interaction between the fibrils [7].
There is tentative indirect evidence that a highly coherent specific axial interfibrillar organisation also exists between collagen fibrils within cornea. In small angle X-ray diffraction studies of cornea, the commonly observed intensity of the meridional diffraction series has a first order intensity that is very weak [8], whereas in most fibrillar collagen rich tissues, the intensity of the diffraction series follows a pattern of strong odd and weak even diffraction peak intensities where the first and third meridional diffraction order of many collagen rich tissues are more intense that the rest of the observable meridional intensities. Such a pattern of intensity in the meridional orders is believed to derive from the well characterised ~65 nm axial repeating step function of the gap and overlap regions that arises from the axial molecular packing of collagen molecule within the fibril the Hodge Petruska model [9] as demonstrated in the schematic in Figure 1.
The difference observed in the diffraction of cornea points to two possible modulations of this structural feature, it is due to either 1) the regular presence of proteogylcans along the fibril that modulates the axial electron density due to collagen axial packing [8], or 2) it is
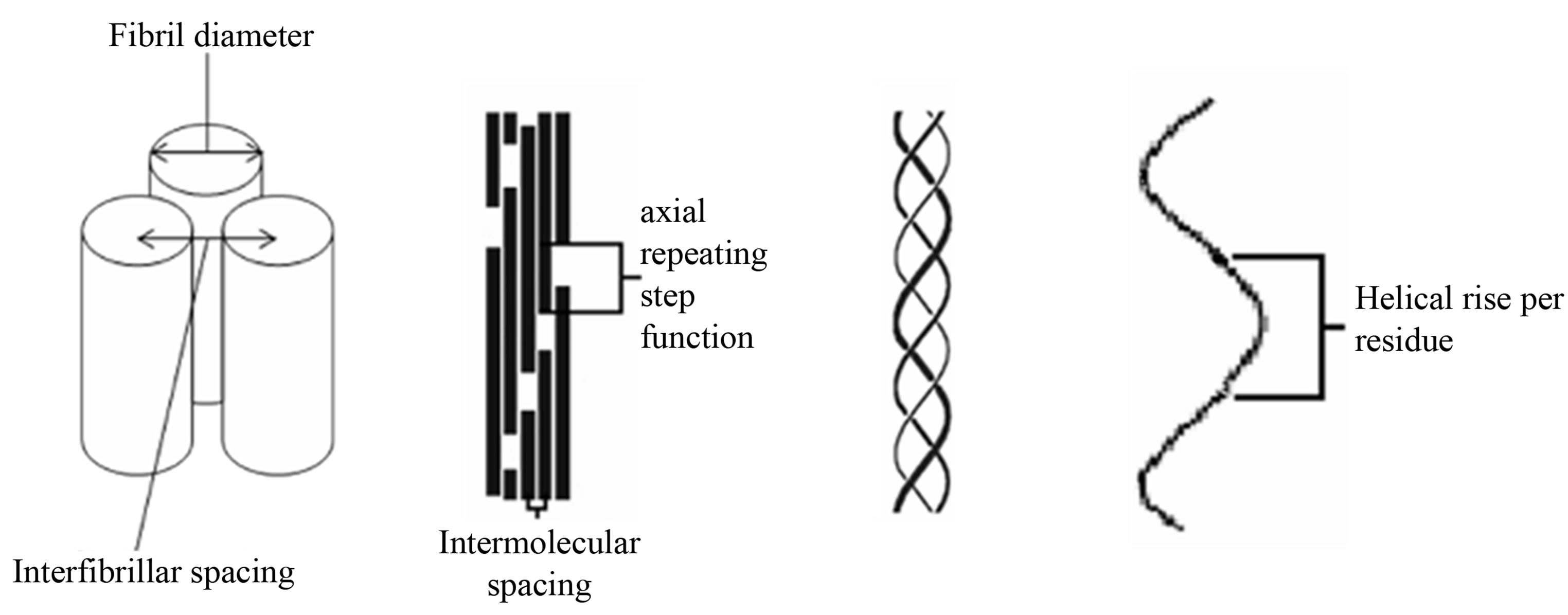
Figure 1. Schematic of the collagen hierarchy. From left to right, fibril packing, microfibril packing, collagen triple helix and helical rise per residue. Diagram is not to scale.
due to a coherent axial suprafibrillar relationship between fibrils that involves a specific axial spatial offset between neighbouring fibrils. Such interactions may be held in place by specific linkages dictated by proteoglycans that are known to be important in the interfibrillar relationships, their spacing, and the all important transparency [5,10,11].
If the second proposition (a coherent axial relationship) here is correct, a possible way of probing the suprafibrillar organisation would be by mechanical extension of the sample whilst simultaneously recording small angle X-ray fibre diffraction data to a point where the suprafibrillar coherent relationship is disrupted and the individual diffraction event from individual fibrils revealed. Here we made a series of mechanical tests on strips of bovine cornea at extensions well above any physiological limit (up to 692% of the rest length) in order to reveal the changes in the interfibrillar relationships that may underlie the resting state.
2. MATERIALS AND METHODS
2.1. Materials
Corneal samples from 6-month old bovine eyes were obtained from a local abattoir and dissected from the scleral tissue leaving approximately 3 cm diameter discs. All samples were dissected from fresh eyes and stored above saline solution to maintain the correct level of hydration. The specimens were cut into 5 mm wide strips from the central portion of the cornea (in case the anatomical position was important, all slices were parallel and sagittal) and mounted onto a specially designed stretching rig. The cornea strips were kept fully hydrated using PBS pH7.4 with an ionic strength of 0.15 M NaCl and subjected to a controlled, incremental strain in the axial direction (up to failure of the sample). Total extension varied between samples due to failure at different strains.
2.2. Data Collection
The X-ray diffraction measurements were conducted at the high brilliance synchrotron source at European Synchrotron Radiation Facility (ESRF) in Grenoble, France on beamline ID02 using a 10m sample-to-detector distance. Small-angle X-ray diffraction patterns were collected simultaneously as the cornea strips underwent applied axial strain in a specially designed stretching rig [12]. Samples were bathed in PBS and covered by two thin mica plates to maintain hydration. Incident X-rays were passed through the centre of each cornea in a direction parallel to the optical axis. Images were collected from 16 different cornea samples on three occasions where the number of images collected varied depending on the point at which the sample failed. Typically between 30 and 40 images were collected during each stretching event on each sample using a Thomson X-ray Intensifier (TH 49-427) lens coupled to a FReLoN CCD camera (2048 × 2048 pixels). This detector has an active area of size 180 mm and a frame rate of 14 images/s (1024 × 1024 pixels), with a 14-bit nominal dynamic range. The delay time between increments of stretch was between 5 and 10 seconds. The exposure time in each case was 0.1 second to minimise dehydration of the cornea. The camera was calibrated using the characteristic 67 nm meridional spacing of rat tail tendon and the wavelength of the X-rays was fixed at 0.0995 nm. The scattering images presented as a series of five meridional diffraction orders superimposed upon a fibrillar interference function and Bessel function due to the size and lateral disposition of adjacent collagen fibrils.
2.3. Data Analysis
X-ray diffraction patterns were analyzed using in house software. The two dimensional detector output was converted into one-dimensional profiles of integrated intensity versus scattering vector Q where
over 360 degrees of isotropy, although in some cases where preferred orientation was observed, different aspects of the scattering image could be extracted. The one dimensional profiles were analyzed using the one dimensional peak fitting software Peakfit4 (AISL).
2.4. Analysis and Identification of Key Reflections
Each X-ray frame contained information from the axial structure of fibrils—the meridional reflectionsscattering from the cylindrical fibrils, interference functions due to the specific distances between fibrils and in some cases unreported long range reflections. Peakfit4 (AISL) software was used to determine the peak size shapes and integrated intensity of the meridional reflections. Peakfit4 (AISL) was used to obtain the peak maxima and minima of the scattering corresponding to the cylindrical transform of the collagen fibrils, these can be well described as a Bessel function where the first Bessel function minimum occurs at
, where R is the reciprocal space coordinate of the reflection and D is the fibril diameter [6].
In addition to calculating the fibril diameter the average centre-to-centre interfibrillar spacing (d) was calculated using d = 1.12 p, where p is the corresponding intermolecular lateral packing Bragg peak [3,6] and 1.12 is a packing factor that assumes the fibrils are arranged in a hexagonal-like lattice.
3. RESULTS
By using a highly intense X-ray beam and recording at very low scattering angles combined with mechanical stretching changes long range order in corneal fibrils can be revealed to a resolution of approximately 200 nm. Our studied showed that the SAXS (Qmax = 0.06 nm−1, min = 0.005 nm−1) pattern of cornea changed significantly with extension and a number of scattering features evolved during extension. We have identified, measured and interpreted the changes observed that speak to changes in interfibrillar interactions.
We have identified, measured and interpreted the changes observed that speak to changes in interfibrillar interactions. Figure 2 shows a gallery of the variety of two-dimensional X-ray diffraction patterns taken from over 400 images of 16 different cornea samples at different stages of mechanical strain. The scattering pattern at resting state shows two main partially superimposed features, firstly a broad peak with a maxim in the region of Q = 0.013 nm−1 that corresponds to the lateral interfibrillar interference function. The radial intensity distribution of this reflection is indicative of the macroscopic organisation of collagen fibrils and fibrillar lamellae, secondly a series of sharper arced meridional reflections where in the resting state the 3rd order at Q = 0.045 nm−1 is prominent. Upon extension, a range of different characteristic features were observed to emerge in the diffraction images, the most important were as below.

Figure 2. Two-dimensional X-ray diffraction patterns collected from different cornea samples at different stages of mechanical strain. (a) A typical image taken from a cornea with no mechanical strain applied to the cornea, (b) shows an image where the first order meridional reflection of cornea is beginning to be observed after application of mechanical strain. (c) Shows an image where a new reflection has appeared which corresponds to a periodicity of approximately 120 nm. (d) An image where preferential alignment is observed in the meridional reflections. (e) Shows a new reflection corresponding to a periodicity of approximately 120 nm. (f) Shows the presence of two distinct interfibrillar reflections corresponding to different interfibrillar distances. (g) and (h) show a more pronounced and visible first order meridional reflection of cornea.
3.1. Emergence of the First Order Meridional Reflection
During strain extensions to breakage-up to 692% percent in the highest case, and in some cases before the sample failed macroscopically, a strong first order of meridional diffraction peak was observed, this is in addition to the third order that is usually the most prominent meridional diffraction in the resting state.
The position and breadth of the refection with respect to scattering vector Q, and therefore the axial lattice structure within the fibrils did not change sensibly. Example X-ray scattering patterns exhibiting this feature are shown in Figures 2(d), and (f)-(h) there are at extensions of 80%, 558%, 650% and 692% of the rest length respectively within different samples.
These clearly show the emergence of a first order diffraction peak at Q = 0.015 nm−1 that is visible above the lateral interfibrillar scattering pattern at Q = 0.014 nm−1. The observation of the first order of meridional diffracttion at the highest levels of extension observed (over 500%) was combined with a much decreased peak corresponding the interfibrillar interference scattering indicating a decoupling and loss of spatial regularity between the fibrils both axially and laterally (Figures 2(g) and (h)).
A corresponding one-dimensional linear trace for cornea upon application of mechanical strain within a single cornea is shown in Figure 3.
Figure 3(a) shows the radially averaged scattering profile from the rest state cornea with no mechanical strain applied. Figures 3(b) and (c) shows the emergence of a first order reflection upon application of mechanical strain at 233% and 675% the rest length and the loss of the lateral interfibrillar interference peak. The presence of the first order reflection is visible at a position of 0.015 nm−1 which corresponds to an axial-period of 66.6 nm this value does not change during the mechanical testing as judged by the movement of the 3rd meridional order which is visible in all samples. The scattering profile in Figures 3(a) and (b) also shows the broad lateral interfibrillar interference function at 0.013 nm−1 and 0.014 nm−1 respectively. This movement possibly confounds the appearance of a weak first order in the resting state. The appearance and minimally altered position of higher orders of the meridional diffraction series demonstrates that the collagen axial periodicity within a fibril remained.